1 Introduction
In the last few decades, the scientific community has eagerly been trying to develop systematic methods to enable engineers to create sustainable products – that is, ‘discrete manufactured products that, in fulfilling their functionality over their entire life cycle, cause a manageable amount of impacts on the environment while delivering economic and societal value’ (Bonvoisin, Stark & Seliger Reference Bonvoisin, Stark, Seliger, Stark, Seliger and Bonvoisin2017). As early as Reference Baumann, Boons and Bragd2002, Baumann et al. identified more than 150 methods for ‘green product development’ – focusing solely on the environmental dimension of sustainability. More recently, Pigosso (Reference Pigosso2012) has identified 106 different approaches, ranging from simple guidelines to complex assessment methods, such as Life Cycle Assessment (LCA).
Due to the multitude of available methods, Ernzer & Birkhofer (Reference Ernzer and Birkhofer2002) state that the difficulty no longer lies in the development of further design methods, but rather in the selection of those methods that can be applied efficiently to a specific situation. As a matter of fact, the existing methodological support for sustainable product development (SPD) is often criticized for being poorly integrated into the product development process (Knight & Jenkins Reference Knight and Jenkins2009; Rosen & Kishawy Reference Rosen and Kishawy2012).
Despite the diversity of the approaches developed in the last years, SPD continues to be challenging. There are neither agreed standards for an SPD process, nor extensive case studies showing the benefits and obstacles of implementing sustainability. Instead of contributing to the further inflation of the pool of unused design methods, what is now needed is a critical reflection on the existing approaches.
This article provides such a reflection by reporting the application of three SPD methods in the context of a concrete product development project. The case to be studied has been chosen from the field of urban mobility – a significant area of human life in social and environmental terms. A 2-year-long product development project has been carried out by the authors to determine:
-
(i) the degree to which the underlying scientific paradigms of SPD can be implemented in a real product development process;
-
(ii) how existing SPD methods can contribute to this challenge.
To answer these questions, a framework is first presented, which consolidates the various aspects to be combined in order for products to be considered as sustainable (see Section 2). The framework provides the theoretical basis for identifying the problem addressed in the case study (what to develop; see Section 3) and for selecting appropriate design methods in the product creation process (how to develop; see Section 4). Section 5 gives a detailed report of the development project itself by describing the applied design methods. The results of this process are then reflected in Section 6 and concluded afterwards.
2 Framework for sustainable product development
While many methods for SPD have been developed, there is no undisputed standard that gives an overview of the elements to consider for developing a sustainable product. Most available methods focus on narrow aspects such as material selection (e.g., GRANTA sustainable development databaseFootnote 1 ) or the comparison of different end-of-life strategies for a product (Gehin, Zwolinski & Brissaud Reference Gehin, Zwolinski and Brissaud2008). To find a common ground for sustainability theory and the case study in this article, the framework depicted in Figure 1 has been developed on the basis of the paradigms commonly discussed in literature.
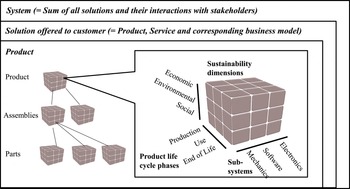
Figure 1. Framework for sustainable product development (based on Buchert, Pförtner & Stark Reference Buchert, Pförtner, Stark, Stark, Seliger and Bonvoisin2017b ).
The cubes in Figure 1 represent an abstract view of a product in a hierarchical structure. They depict parts or overarching assemblies and can be characterized into three dimensions:
-
(i) The product life cycle and its phases – from production over the usage phase until the end-of-life phase. As a basic prerequisite for SPD, all phases need to be considered in conjunction.
-
(ii) The three sustainability dimensions, namely the social, economic and environmental effects of design decisions.
-
(iii) The subsystems of a product show the area of influence of design engineers on the final outcome by defining the mechanics, electronics and utilized software of the product.
Combination of these three dimensions forms “problem modules” within a cube. Every module constitutes an own topic in the context of SPD, for example, economic effects of retrofitting outdated machines via software updates. Another example could be environmental assessment of manufacturing mechanical parts of an electric motor. The challenge concerning SPD is that an isolated view on respective problem modules only leads to a local optimum. Changes conducted for one module propagate through the system and often result in target conflicts and trade-offs. Hence, decision-making in SPD should be seen as a multi-attribute optimization problem.
The consequences of design decisions need to be monitored and validated at all hierarchy levels of the product structure depicted in Figure 1. At the part level, validation involves the consideration of material decisions, for example. At the assembly level, the overarching parameters that result from the combination of different parts (e.g., motor efficiency) are included. At the product level, the interaction with the user needs to be considered, such as by user comfort, functionality, or safety aspects of the considered product.
In addition to the product itself, there is a consensus that the overall solution offered to the customer – in which the product is embedded – needs to be considered. This requires not only consideration of the product but also the underlying services and business models (see for example McAloone & Pigosso Reference McAloone, Pigosso, Stark, Seliger and Bonvoisin2017).
Furthermore, the scope should be extended even more to evaluate how the solution performs against competing products, or how the substitution/modification of one system parameter affects the overall sustainability performance at the system level (see, for example, Azevedo et al. (Reference Azevedo, Bras, Doshi and Guldberg2009) or Ceschin & Gaziulusoy (Reference Ceschin and Gaziulusoy2016), for a specific focus on mobility).
This framework provides a conceptual basis for the development of more sustainable products, which can be applied to all technical product types. However, as the framework is described at a high level, it is not adequate for operational decision support in the design process. The following case study evaluates how selected methods for SPD can contribute to this challenge. The study addresses the field of urban mobility, which has a large impact on public health as well as on the environmental and economic sustainability dimensions.
The following section presents an initial analysis of the mobility system, as the overarching layer of the framework illustrated in Figure 1. It outlines major problems in the context of urban mobility and discusses the advantages and drawbacks of possible solutions. In particular, the potentials of pedelecs are outlined and it is discussed why the diffusion of this mobility option is still inadequate in urban areas.
3 Case study motivation and background
Lam & Head (Reference Lam, Head, Inderwildi and King2012) highlight major areas of concern regarding the sustainability of existing mobility systems:
-
(i) They account for 23% of worldwide greenhouse gas (GHG) emissions. Transport-related GHG emissions increase faster than any other energy end-use sector (IPCC Reference Edenhofer, Pichs-Madruga, Sokona, Farahani and Kadner2014).
-
(ii) They contribute to poor air quality. Exposure to outdoor particulate matter causes 3.2 million deaths per year worldwide (Lim et al. Reference Lim, Vos, Flaxman, Danaei, Shibuya, Rohani and AlMazroa2012).
-
(iii) In Germany, the USA and the UK, traffic-related pollution accounts for 20% of overall particulate matter in the atmosphere (Jerrett Reference Jerrett2015).
-
(iv) Economic impacts related to the rising fuel cost, as well as time wasted in traffic congestions, are becoming increasingly relevant.
Different approaches have been introduced to address these challenges. Holden (Reference Holden2012) summarizes these approaches by advocating for the reduction of the overall traffic volume, the improvement of energy and material efficiency of mobility systems, and a transition from systems based on fossil fuels to those based on renewable energy.
A reduction in the overall traffic volume can be seen as a beneficial solution, but it seems unrealistic in the light of recent traffic statistics. In Germany, for example, the annual travelled distance increased from 559 to 627 billion kilometres between 2000 and 2014.Footnote 2 Hence, improvements in efficiency and the development of new technologies were the focus in the last few years, for the automotive industry in particular. Since the efficiency of combustion engines is subject to certain physical limits, alternative optimization potentials are currently exploited, ranging from lightweight design to driver assistance systems. Regarding the transition to renewable energies, alternative drivetrain technologies are currently being developed. In this context, there is a broad discussion on the mitigation potential of electric mobility. Electric vehicles provide a certain potential to reduce (carbon-based) emissions in their usage phase (Canals Casals et al. Reference Casals, Martinez-Laserna, García and Nieto2016). Indeed, some studies, such as the one by Hawkins et al. (Reference Hawkins, Singh, Majeau-Bettez and Strømman2013), show that electric cars can achieve a reduction in specific environmental impacts, in particular the global warming potential. Hence, there is encouragement at the political level for the diffusion of electric cars to achieve national emission targets. For example, Germany strives for one million electric cars by 2020Footnote 3 and has recently started subsidizing electric car purchase.Footnote 4 Despite the potential reduction of emissions, the overall positive effects of electric cars have been questioned by several authors, as the mitigation of environmental impact highly depends on the energy mix used for charging the battery. Furthermore, electric car production leads to a larger environmental burden in comparison to fossil-based passenger cars, with battery production as the major influencing factor (Hawkins et al. Reference Hawkins, Singh, Majeau-Bettez and Strømman2013). The adoption of electric cars also relies on a change in the whole mobility system, including the necessary surrounding infrastructure, such as charging stations (Augenstein Reference Augenstein2015).
Complementary to electric cars, the invention of pedal electric cycles (pedelecs) – which integrate an electric motor into the bicycle design – can play a role in substituting fossil-fuel-based vehicles. In comparison to small passenger cars, pedelecs exhibit lower production and use-phase burdens. Furthermore, health benefits and time saving in dense traffic can be achieved. Their smaller size also increases the available urban space (Rudolph Reference Rudolph2014). In comparison to bicycles, electrically supported cycling increases the cycling comfort and addresses a broader range of target groups. For instance, pedelecs allow users to travel larger distances by decreasing cycling efforts, especially in hilly environments. Moreover, pedelecs can be suitable for people with disabilities, who may not be able to make use of conventional bike mobility. In 2006, more than 14 million two-wheeled pedelecs were sold in China, which equals the number of gasoline-powered two wheelers sold in the same period (Weinert et al. Reference Weinert, Ogden, Sperling and Burke2008). The high diffusion rates in China can be explained by the low cost of pedelecs in comparison to cars. Furthermore, in 2006, 148 Chinese cities imposed regulations for banning motorcycles (Weinert et al. Reference Weinert, Ogden, Sperling and Burke2008). In this context, Creutzig, Mühlhoff & Römer (Reference Creutzig, Mühlhoff and Römer2012) add that policy interventions in favour of cycling could also be relevant for European cities. They show how future decarbonization policies could lead to a significant increase in cycling, particularly in the smaller cities. A share of more than 50% by 2040 can be considered realistic (Creutzig et al. Reference Creutzig, Mühlhoff and Römer2012). Currently, cycling and e-bike transportation ranges from less than 1% (e.g., in Cagliari, Italy, 2001) to 30% (e.g., in Groningen, the Netherlands, 2009) in European cities.Footnote 5 A bike mobility share of this magnitude implies that a large number of cars and motorcycles need to be substituted. In this context, the question of whether existing solutions for cycling are sufficient is relevant. In particular, it needs to be evaluated why pedelec diffusion is so low in European countries without policy intervention. Currently, only 3.4% of all households in Germany own pedelecs, adding up to a total of approximately two million units.Footnote 6
The existing initiatives for increasing bike adoption, such as sharing schemes (for example, see Paul & Bogenberger (Reference Paul and Bogenberger2014)) or more secure bike lanes, provide the necessary boundary conditions for transformation. However, several aspects hinder the broad implementation of urban cycling in Germany and other western countries. The physical effort associated with conventional bicycles discourages many people (Rose Reference Rose2012). Furthermore, common bicycles are too dependent on the surrounding climate. The latter point also applies for current pedelec designs. Pedelecs lack essential features normally expected by car users, such as protection from rain or transportation of cargo. Furthermore, pedelecs are often designed as ‘bicycles with motors’, evoking prejudices and sceptical attitudes (Le Bris Reference Le Bris, Wulfhorst and Klug2016). The perceived obstacles to pedelec implementation (e.g., high price, greater weight) also indicate that customers see current pedelecs as more of an equivalent to bicycles than to cars (Prill & Lanzendorf Reference Prill and Lanzendorf2012).
Different concepts for enhancing the capabilities of human-powered urban transport have been developed in the last years. Cox (Reference Cox2008), for example, introduces different bicycle concepts for increasing the diffusion of bike mobility, such as foldable bikes, cargo-bikes or velomobiles. More recently, the ‘Electric Persuasive Vehicle’ approach from MITFootnote 7 presents a new concept for utilizing pedelecs in urban mobility.
While these concepts address the need for more sustainable mobility at the system level, an optimization of the product itself in terms of sustainability has not been attempted. The following case study presents an attempt towards a more holistic view of sustainable mobility, by adhering to the framework shown in Figure 1.
4 Case study approach
In this section, the performed case study, its mission and the corresponding project setup are described in detail. Selected SPD methods are introduced and their utilization in the case study is described.
4.1 Case study mission
Based on the results of the initial analysis presented in the last section, the underlying hypothesis of the product development project is that an increased rate of kilometres covered with pedelecs would be beneficial for the overall sustainability of urban traffic. In this context, the limited functionality of pedelecs in comparison to cars is identified as one of the main barriers to pedelec diffusion. Hence, improvements on mobility system level (see Figure 1) can be achieved by enhancing functionality for substituting small passenger cars for short and medium distances, for example, for commuting or grocery shopping. For addressing the other layers of Figure 1, the pedelec itself, its components and the corresponding services are considered in the scope of the development project.
4.2 Case study setup
The product development project has been conducted in the timeframe of two years (2013–2015), from the initiation phase up to the fabrication of a fully functional prototype. It involved two design engineers, a manufacturing engineer, two sustainability experts and a management board. The design engineers played two different roles in the project. The technical chief coordinated the work on product parts or assemblies and organized project planning activities, while the quality manager ensured that the product properties matched the specifications, performed the budget planning and participated on strategic concept decision-making. In addition, the sustainability experts provided background knowledge on sustainability considerations and performed specific tasks related to sustainability assessment. Some members of the project team were regular cyclists and some commuted to work in their private car. Hence, the customer perspective could be also discussed within the project team. Regular meetings were organized between the design team and the sustainability experts to discuss the impacts of strategic design decisions in terms of sustainability effects and manufacturability (see also. Buchert et al. (Reference Buchert, Steingrímsson, Neugebauer, Nguyen, Galeitzke, Oertwig, Seidel, McFarland, Lindow, Hayka and Stark2015) for a focus on the interdisciplinary team setup and other associated projects). The project success was monitored in terms of achievement of the requirements in regular meetings with the management board.
4.3 Selection of design methods
The available approaches for SPD can be classified into different categories (Baumann et al. Reference Baumann, Boons and Bragd2002). Checklists and guidelines provide a generic support for sustainable product design based on best practices. A large variety of guidelines are available, in particular addressing environmental sustainability (see Telenko et al. Reference Telenko, O’Rourke, Seepersad and Webber2016). Analytical assessment methods such as Life Cycle Sustainability Assessment (LCSA, see Finkbeiner et al. (Reference Finkbeiner, Schau, Lehmann and Traverso2010)) provide a framework for assessing the sustainability impacts of products from a theoretical perspective. Rating and ranking approaches (such as the Product Sustainability Index [ProdSI] developed by Shuaib et al. (Reference Shuaib, Seevers, Zhang, Badurdeen, Rouch and Jawahir2014)) are simple tools that help to compare different alternatives regarding predefined criteria, for example, energy consumption of production processes or the amount of material needed.
As all method categories have advantages and disadvantages a combination of different methods seems reasonable. In the scope of previous work, a comprehensive approach for selecting and combining design methods focusing on at least one of the sustainability dimensions was developed (Buchert et al. Reference Buchert, Halstenberg, Bonvoisin, Lindow and Stark2017a ). The developed taxonomy and corresponding method database was utilized to test applicability of different methods in the context of ecodesign and also sustainability on the example of the pedelec. For the present case study, three methods are introduced which adhere best to the specific focus given by the framework in Figure 1 (consideration of all three sustainability dimensions and all stages of the product life cycle). Only few methods were identified as suitable for these specific requirements. A more detailed justification for selection of respective methods is given in the description of selected approaches below.
4.3.1 Design for Sustainability Strategies (DSS)
Only two guidelines considering all three sustainability dimensions could be identified (see also Byggeth, Broman & Robèrt Reference Byggeth, Broman and Robèrt2007). The DSS was chosen as it is based on the UNEP Ecodesign Checklist (Brezet et al. Reference Brezet, Hemel, Böttcher and Clarke1997) which is already widely recognized in the field of Ecodesign. It was updated by Crul & Diehl (Reference Crul and Diehl2006) with extensions in regard to economic and social factors (see Table 1). The strategies focus on different phases of the product life cycle.
Table 1. Design for Sustainability Strategies, based on Crul & Diehl (Reference Crul and Diehl2006)
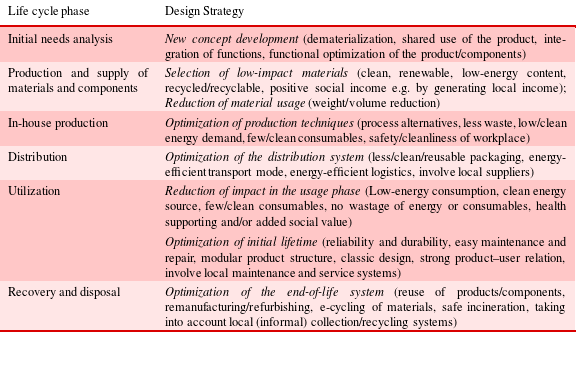
4.3.2 Simplified Life Cycle Sustainability Assessment
LCSA refers to the integration of three methods – LCA, Life Cycle Costing (LCC) and Social Life Cycle Assessment (SLCA), as defined by Kloepffer (Reference Kloepffer2008) and Finkbeiner et al. (Reference Finkbeiner, Schau, Lehmann and Traverso2010). It was included in the study as it can be considered as the most mature approach for an integrated quantitative assessment of the environmental, social and economic implications of a product along its product life cycle. It is also driven by the initiatives UNEP and SETAC and a network of researchers which aim at the development of a standard for sustainability assessment (see Ciroth et al. Reference Ciroth, Finkbeier, Hildenbrand, Klöpffer, Mazijn, Prakash, Sonnemann, Traverso, Ugaya, Valdivia, Vickery-Niederman, Valdivia, Ugaya, Sonnemann and Hildenbrand2011).
Due to the high ambitions of this methods, the practical use and scientific robustness of LCSA still face some challenges. As an alternative to this extensive method, Neugebauer et al. (Reference Neugebauer, Martinez-Blanco, Scheumann and Finkbeiner2015) introduced a simplified approach called the ‘sustainability footprint’, which enables a straightforward assessment by including a limited number of indicators, namely global warming potential, fair wages, and value added. The indicators have been chosen on the basis of a criteria-based ranking providing a hierarchical selection of topics in the LCSA context. The global warming potential indicator is generally acknowledged as robust by the scientific community and is thus widely implemented. On the other hand, robust methods addressing fair wages and value added are still missing. In the following section, the best available indicators provided by Neugebauer et al. (Reference Neugebauer, Emara, Hellerström and Finkbeiner2015) are used. Furthermore, suggestions for calculating a product’s value added are followed for economic perspective on LCSA (see Neugebauer, Forin & Finkbeiner (Reference Neugebauer, Forin and Finkbeiner2016)).
In comparison to the DSS – which aims at supporting the synthesis of new designs by providing heuristics – LCSA targets the determination of concrete impact through the generation of quantitative descriptive results.
4.3.3 Product Sustainability Index
The ProdSI, introduced by Shuaib et al. (Reference Shuaib, Seevers, Zhang, Badurdeen, Rouch and Jawahir2014), belongs to the category of rating and ranking tools and includes a set of 47 criteria for evaluating products, considering different life cycle phases and the three sustainability dimensions. Only one comparable method in this category was identified (the Bournemouth University Model, see Howarth & Hadfield Reference Howarth and Hadfield2006). The ProdSI was chosen in favour as it is based on a comprehensive review of available criteria sets for assessing and comparing environmental, economic and social implications of a product design. Assessment results are then aggregated to a score or can be expressed in a spider-web diagram (see Figure 2). While LCSA strives to a general assessment of environmental, social and economic impacts suitable for products or processes, ProdSI provides a more customized set of criteria for analysis of products by also taking into account parameters that describe the product quality and durability, for example.
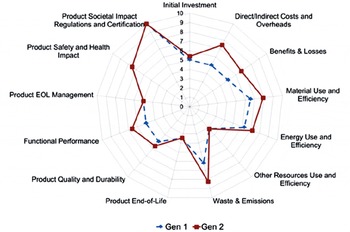
Figure 2. Exemplary ProdSI for two product generations (Shuaib et al. Reference Shuaib, Seevers, Zhang, Badurdeen, Rouch and Jawahir2014).
4.4 Case study overview
This section provides an overview of the sustainability-related activities conducted at different decision points of the design process. Figure 3 displays an overview of the different steps and the case studies involved. The different boxes represent the aspects that have been addressed by applying SPD methods in different project phases. Therefore, the inclusion of various sustainability aspects into the product design has been ensured. The selected methods are particularly applied in the early phases of the product creation process (clarification of the task and conceptual design), since the influence of decisions on the properties of the final product is at the highest in this phase. The following paragraphs explain the motivation for and connections between the different sustainability-related activities. The concrete outcome of these activities and the main results of the case study are part of Sections 5 and 6.
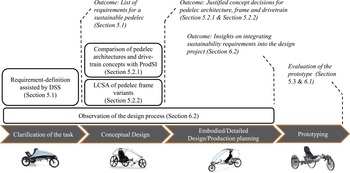
Figure 3. Utilized methods in the case study.
4.4.1 Clarification of the task (requirement definition)
As a basis for developing an improved product concept, a list of requirements (see Section 5.1) is presented, which addresses the necessary functionalities for increasing customer appreciation. To support this step, the Design for Sustainability strategies are applied to address improvements in the mobility product as a sum of its assemblies and components.
4.4.2 Conceptual design
Following the general product planning, an initial conceptual design of an adequate pedelec solution is targeted. This design phase focuses on subsystems and parts of the product under consideration. For this purpose, the product architecture, the drivetrain and the pedelec frame were focused upon. These subsystems/components were chosen because material production and energy consumption in the usage phase are the most significant levers for the improvement potential on product level (Neugebauer et al. Reference Neugebauer, Chang, Maliszewski, Lindow, Stark and Finkbeiner2013). Concerning the product architecture and drivetrain, various advantages and disadvantages of different pedelec designs are collected, and compared in terms of the criteria based on the ProdSI (see Section 5.2.1). The different material options of the pedelec frame are assessed through the previously described LCSA method. The results can be found in Section 5.2.2.
4.4.3 From clarification of the task until prototyping
Complementary to the described design activities, the product creation process has been observed and reflected by the project team with a specific focus on applied methods and implications on the decision-making process in the project. The insights derived in this context are presented in Section 6.2.
5 Design of a new mobility solution
This section describes the course of the sustainability-related activities performed in the development process, highlighted in the previous section. The results of the application of the three selected SPD methods are described. Finally, the outcome of the development process – a pedelec termed the Smart Urban Wheeler (SUW) – is presented.
5.1 Method application in the phase ‘clarification of the task’
In order to achieve the case study mission, a list of requirements is presented in this section (see Table 2). The first four requirements (R1–R4) were defined to enhance pedelec functionality through attributes like comfortable seating, cargo transportation options, passenger seats for children and weather protection. In addition to this targeted systemic change in the mobility system, the product itself has to follow common sustainable principles, as defined by the Design for Sustainability Strategies (see Section 4). The strategies are used to find improvement potentials on the basis of influential phases of the pedelec life cycle, that is, energy consumption in usage and material production in particular (Neugebauer et al. Reference Neugebauer, Chang, Maliszewski, Lindow, Stark and Finkbeiner2013). These strategies were used to identify R5–R15. Four strategies are therefore combined: (1) selection of low-impact materials, (2) reduction of material usage, (3) optimization of initial lifetime and (4) reduction of impact in the usage phase. The first two strategies were adapted and directly included in the requirement list (R5 and R6), as they describe straightforward aspects of product design, which can be influenced at a very early stage of the product development process. R5 determines how material is produced by using the least amount of resources possible for fulfilling the given function. R6 reflects a reduction in material usage by utilizing lightweight materials, since the weight of a vehicle has a significant influence on its sustainability performance in the usage phase. The third strategy (optimization of initial lifetime) needs further consideration, as it addresses the question of how to make best use of the first usage period of the product. When addressing the initial lifetime of a pedelec, the aspects of modularity and adaptability play an important role in customizing the new mobility solution to the individual needs of different customer groups. Therefore, a modular rack allows the transporting of different equipment (e.g. a child restraint or a luggage box) (R7). In addition, a modular drive concept is included for enabling flexible power requirements (R8). The fourth strategy is addressed in multiple ways. For reducing the energy consumption during usage, a solar panel is included in the mobility concept. The energy concept also includes the required infrastructure for home-based battery charging, which does not directly reduce the grid-based energy needed, but allows the adoption of renewable energy concepts, depending on the end-user’s preference (R9/10). Furthermore, battery charging through pedalling by means of a generator is considered (R11). In addition, the recuperation of braking energy prevents further energy losses (R12).
Table 2. Requirements for pedelec development with a focus on sustainability
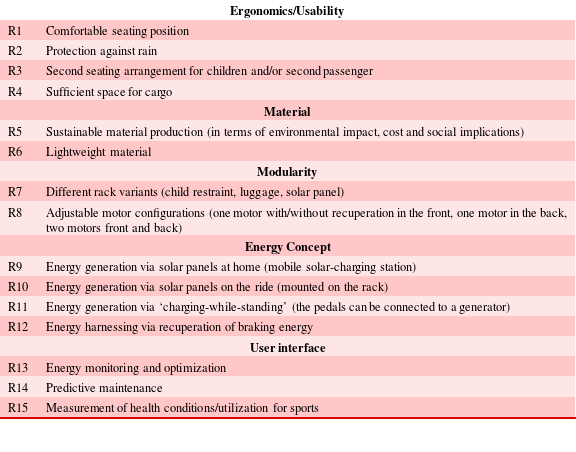
The last two strategies shall also be supported by a user interface, in particular by providing information on energy consumption of the pedelec and advice for driving (R13). Furthermore, maintenance notifications are displayed for prolonging the pedelec’s lifetime (R14). Finally, health-tracking while exercising allows for social considerations (R15).
Based on this list of specifications, decisions on how to implement the new design concept can be made. This includes the selection of suitable suppliers, but also decisions on redesigning specific parts, for example, the frame, the steering and seating systems, and the drivetrain concept. The details of the resulting conceptual design are presented in the next section.
5.2 Method application in the phase ‘conceptual design’
Based on the specifications presented in Table 2, the conceptual design phase includes decisions concerning the product architecture, drivetrain concept and frame material.
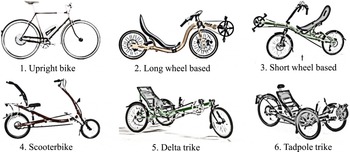
Figure 4. Pedelec architecture types.
5.2.1 Comparison of pedelec architectures and drivetrain concepts
For specifying the underlying pedelec architecture and the drivetrain concept, specific criteria are defined in the first step. The utilized criteria are based on the given set of the ProdSI (see Section 4). On the lowest level of aggregation, the ProdSI comprises 47 different indicators (Shuaib et al. Reference Shuaib, Seevers, Zhang, Badurdeen, Rouch and Jawahir2014). As a discussion of mobility concepts with this many indicators would exceed the scope of this article, the indicators are classified into six categories – emissions, resource demand, ergonomics/comfort, functionality/quality, safety/reliability and cost. While the first, second and last categories are also addressed by means of the LCSA (exemplarily shown for the frame concept), the rest is solely considered within the ProdSI. Both the pedelec architecture and the drivetrain concept are evaluated on the basis of the defined criteria. Since the primary target of the design project is to enhance pedelec functionality for substituting small passenger cars, the factors with the highest prioritization are safety, comfort and functionality.
Table 3. Qualitative comparison of trike designs
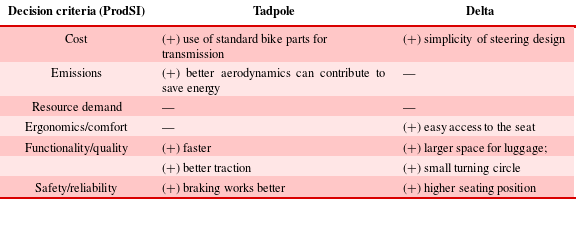
Pedelec architecture
In the history of bicycle and pedelec design, various bike architectures have been developed. To determine which architecture type is most suitable for the targeted mobility product, the existing pedelec and bike concepts have been screened in order to identify the major differences and evaluate their general suitability to the requirements formulated in Table 2. The most promising candidates are then compared on a more detailed basis with ProdSI. Figure 4 gives an overview of the available bike architectures. Upright bikes (1) are the most common form of electric bicycles. However, they have shortcomings in terms of seating comfort and may not be suitable for longer distances in daily commuting for most people. Recumbent bikes (2–6) distribute the weight of the driver across a larger surface and can, therefore, be considered as ergonomically beneficial. Furthermore, recumbent bikes are more aerodynamic, which contributes to the energy-saving strategy addressed in Table 2.
Another decision criterion in this context is the wheel setup which can have two wheels (bicycle) or three wheels (tricycle). Recumbent bicycles (2–4, short- and long-wheel-based bikes as well as scooter bikes) provide less stability and comfort in comparison to trikes, and protection against rain is more difficult to implement. Therefore, trikes are seen as more suitable to the requirements defined in Section 5.1.
Further, considering tricycles, the main differentiation in delta and tadpole tricycles is discussed by the project team in order to identify the advantages and disadvantages of both concepts. Table 3 provides a summary of the main results. In regard to costs, tadpole tricycles have an advantage, as standard bicycle parts for energy transmission can be used. In contrast, delta designs require a cost-intensive differential. Yet, delta tricycles use a less complicated – and therefore less costly – steering design due to the single front wheel. In regard to emissions and energy consumption, tadpole tricycles are more aerodynamic. This may provide benefits in driving speed and energy consumption. Furthermore, the tadpole design provides a better traction due to the electric motor assembly in the back.
Delta tricycles offer easy seat access and larger luggage space behind the seat, which contributes to a higher comfort in comparison to the tadpole tricycle. Furthermore, the delta design provides a small turning circle, which is favoured by the weight distribution across the rear wheels. In regard to safety, a brake on the front wheel provides more braking power than a back brake. Tadpole tricycles typically have brakes on both front wheels and may therefore provide more braking power. However, the driver of a delta may have safety benefits due to the higher seating position. Based on the results presented in Table 3 and the specifications of Section 5.1, the delta tricycle design is chosen for further consideration. Targeting a new mobility solution for urban areas, the high seating position, in combination with the small turning cycle, provides a key advantage. Furthermore, larger luggage space favours the substitution of car transport in terms of work travel or grocery shopping.
Drivetrain concepts
Another design decision discussed in the following paragraphs is the selection of a drivetrain concept. A major challenge in this context is to integrate the ‘charging-while-standing’ requirement (R12) into the design. In a brainstorming session involving both designers of the project team, three configuration options for the drivetrain were defined (see Figure 5).
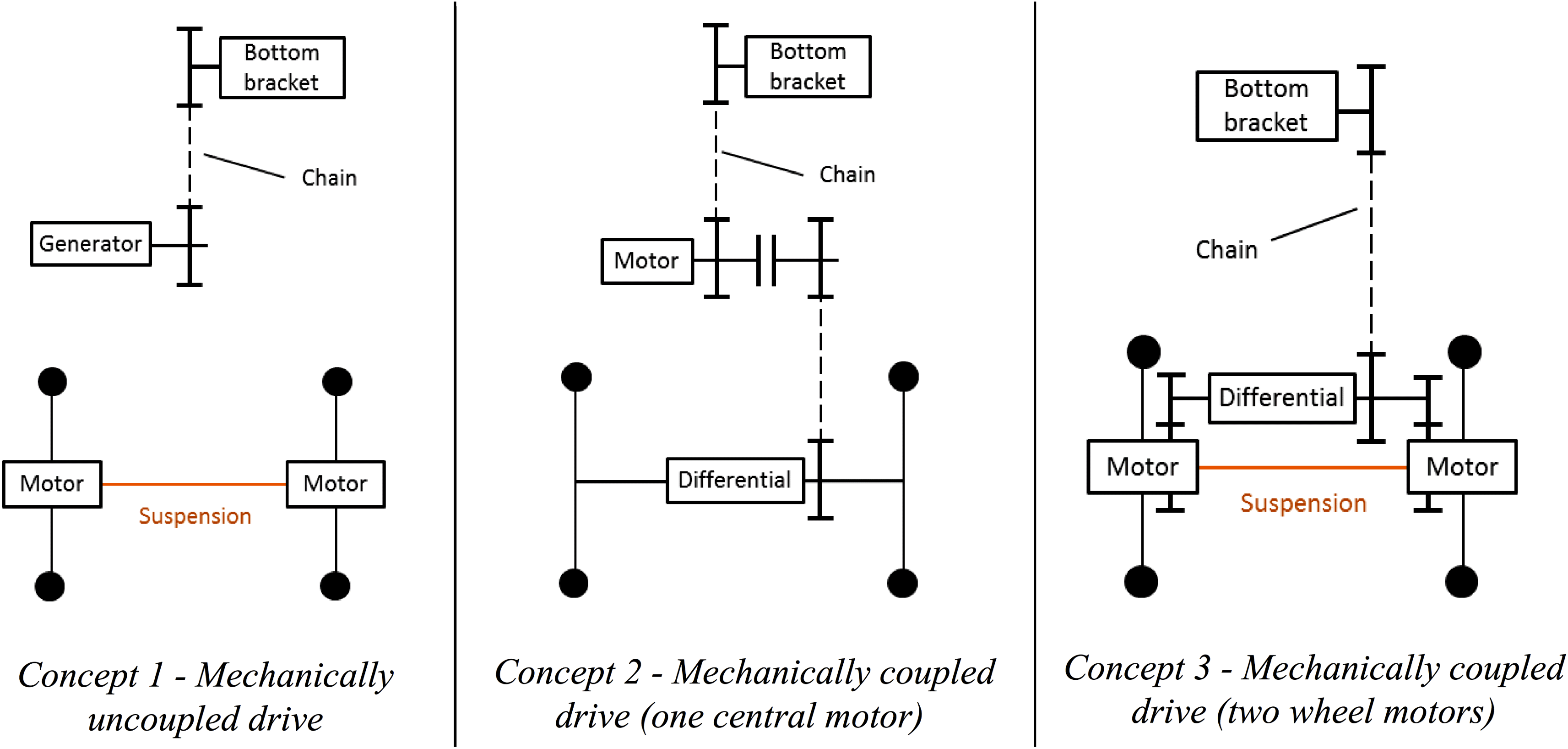
Figure 5. Concept variants for the pedelec drivetrain.
To compare the concepts, the ProdSI framework was utilized again. For better comparison of the three concepts, a spider-web visualization is utilized instead of a table, displayed in a spider-web diagram (see Figure 6). The scale of 1–10 is a result of qualitative evaluation of the concepts in the design team. In addition to the factors introduced above, ‘integration effort’ and ‘technical risk’ are also considered as important decision criteria, since the project needs to adhere to a strict project plan and limited capacities. Since all three concepts provide the same functionality concerning power supply, acceleration and maximum speed the criterion was detailed to ‘driving range’ and ‘innovativeness’.
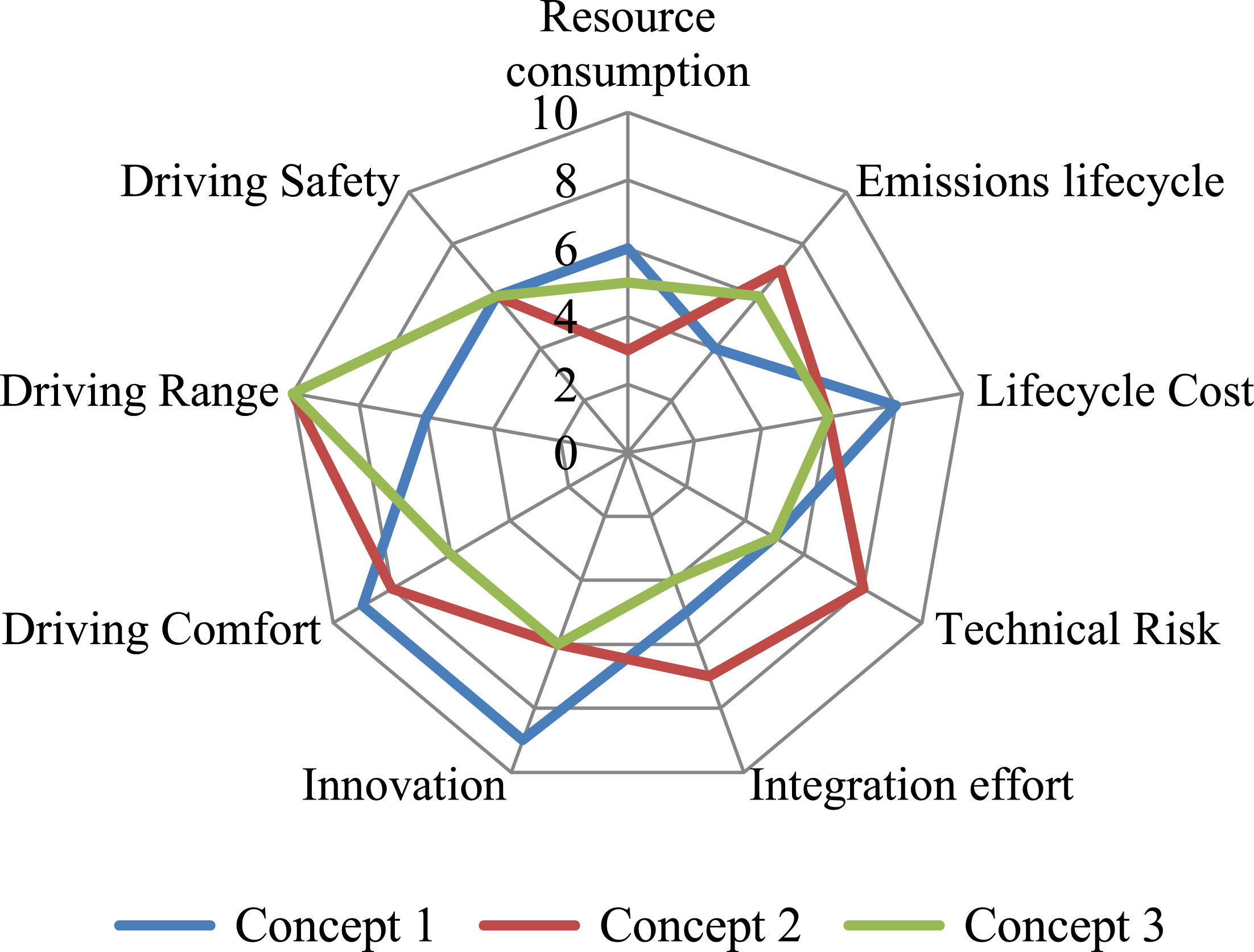
Figure 6. Qualitative comparison of drivetrain variants with ProdSI.
The main idea behind the mechanically uncoupled drive is the missing physical connection between the bottom bracket and the back wheels, which are driven by hub motors. The motors are supplied with energy via a generator connected to the bottom bracket. The main advantage of this principle is that the pedal movement and electric support of the motor can be decoupled, thus facilitating mechanical power transmission. The function of the differential can be realized by motor control. Furthermore, independent wheel suspension can be easily achieved for enabling high driving comfort especially in curves. The ‘charging-while-standing’ requirement can be realized without additional components, leading to lower cost and resource consumption in the manufacturing stage. Furthermore, less maintenance is required due to fewer moving mechanical parts. Nevertheless, due to the innovativeness of this solution, there is a high risk of failure in concept development and implementation. Furthermore, the implementation effort – including tests and electronic steering – is high. Another important disadvantage of the concept in terms of emissions and resource consumption is the low-energy efficiency – estimated at 50–60% – due to losses in converting mechanical energy to electrical energy and converting electrical energy back to mechanical energy. In addition, the battery is highly strained by an alternating direction of energy, leading to lower battery lifetime. If the battery is discharged, the journey can only be continued very slowly, since the wheels cannot be turned manually.
The mechanically coupled drive with one central motor uses a lay shaft with mounted clutch. This clutch can uncouple the back wheels from the remaining drivetrain at traffic lights. Hence, the motor can be utilized as a generator in order to implement the charging-while-standing requirement. Therefore, no additional generator is required. If the battery is discharged, the pedelec can still be moved by pedalling and the battery can last a longer time. Technical risk and implementation effort are lower compared to the first solution option. Nevertheless, the concept is less innovative than Concept 1 and requires components for mechanical transmission (chain, differential, crank, lever for shifting the clutch), leading to a higher weight and maintenance effort.
Concept 3 is also mechanically coupled, but utilizes two hub motors connected to the mechanic drivetrain. Therefore, a sprocket wheel is energized by an additional axis, which has to be mounted on both hub motors. In contrast to the second concept, a generator is necessary to realize the ‘charging-while-standing’ requirement.
Furthermore, switching between normal drive mode and ‘charging-while-standing’ is more complicated. Both hub motors need to be modified to be made attachable to a crank. This adjustment is costly, since customized adaptors are necessary.
The comparison of all three concepts with ProdSI indicates that Concept 2 is more favourable for being implemented into the concept of the SUW.
Table 4. Reference flow values for the different frames considered for the new tricycle concept, including weights, materials and connection/finishing options
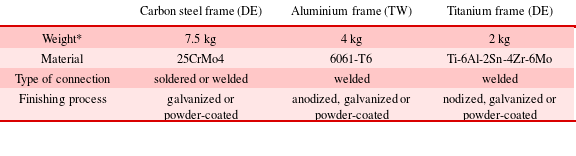
*The weight was estimated with a simplified calculation based on material’s density and different wall thicknesses based on the material’s tensile strength.
5.2.2 Simplified LCSA of pedelec frame variants
In regard to the required redesign of a usual pedelec frame, three technically appropriate materials – steel, aluminium and titanium – have been identified by the design team. These three frame materials are compared in terms of their sustainability performance by means of a simplified LCSA. The chosen indicators of this so-called sustainable footprint are introduced in Section 4.3.2, targeting the inclusion of all three sustainability dimensions from the very beginning. For the LCSA, all relevant processes are included – beginning from the raw material extraction via transport processes and ending with the frame production step. While the system boundaries for the environmental and social dimensions are identical, the economic dimension additionally includes the distribution stage, in order to determine purchase prices and thus calculate the value added. Different production and finishing options are considered for the different frames (see Table 4), which are relevant from an economic and environmental perspective, but hardly cause any difference to the social considerations.
In accordance with the current market situation, it is assumed that the aluminium frames are produced in Taiwan, whereas the steel and titanium frames are produced in Germany. The raw materials are supplied from different countries. The iron ore is derived from Brazil (Triebskorn Reference Triebskorn2012), the bauxite comes from China (BGR 2013) and the titanium ore is supplied by Russia (Drobe & Killiches Reference Drobe and Killiches2014). The system boundary is defined accordingly. The functional unit for the comparative assessment is set to one piece of frame. This leads to different reference flows in regard to the required material amounts, connection types and finishing processes for the different frames (compare Table 4).
Simplified life cycle assessment results/environmental sustainability footprint results
For the environmental dimension, the global warming potential is calculated by considering three main process steps – the raw material production, the transportation and the frame production. Therefore, the GaBi databaseFootnote 8 and the CML methodFootnote 9 are used.
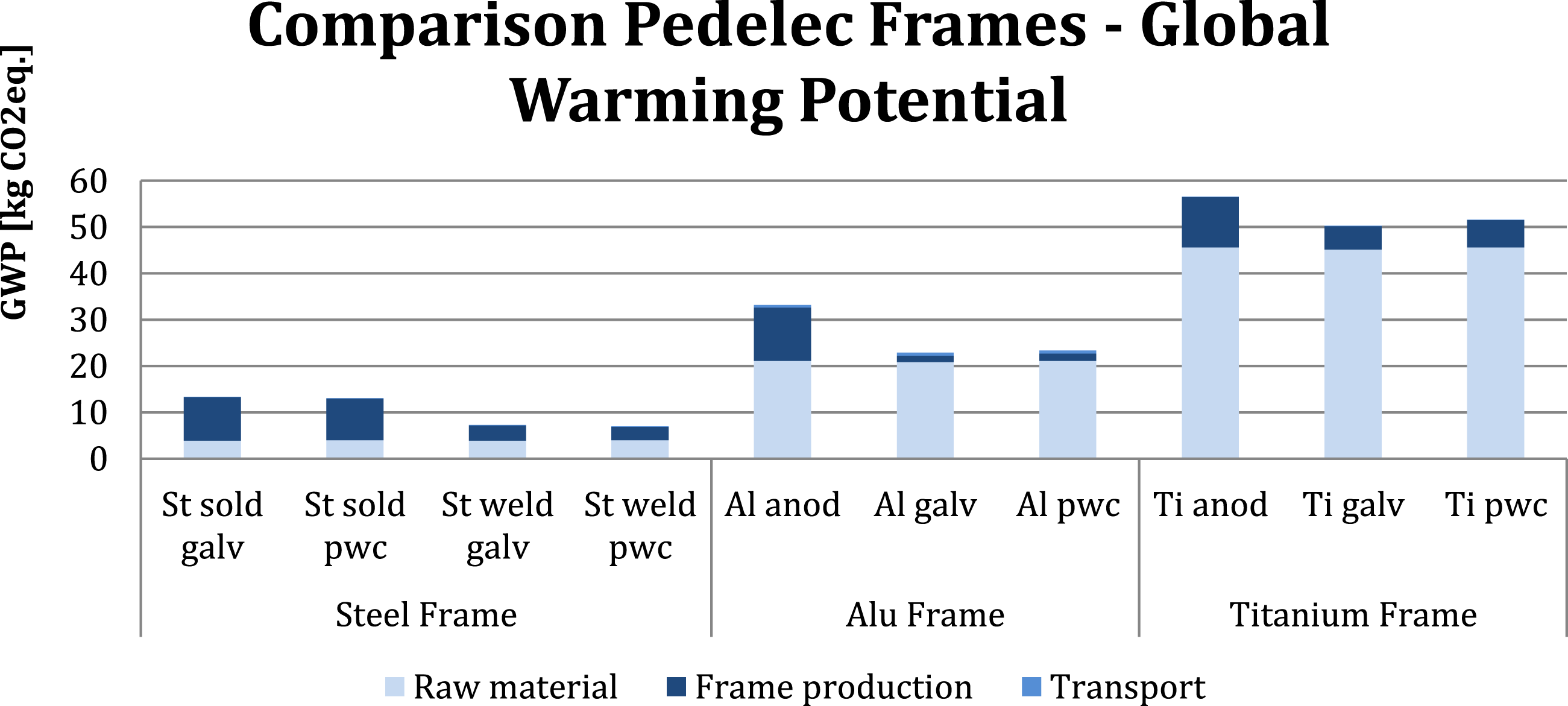
Figure 7. Global warming potential for the tricycle frame options; the results display the reference flow-related requirements of Table 4.
As shown in Figure 7, there are great differences between the different frame materials. The titanium frame performs worst, as its raw material production, with
${>}45~\text{kg}~\text{CO}_{2}\text{eq.}$
, has a high impact. The aluminium frame has a lower raw material burden than the titanium frame. The carbon steel frame has by far the best performance, as its raw material production undercuts that of the other materials.
Simplified social life cycle assessment results
The fair wage potential (FWP) is chosen as an indicator for the social sustainability dimension (compare Section 4.3.2). It is focused on the groups of workers who are expected to contribute the greatest share of working hours, such as manufacturers, miners and transportation workers. This includes – according to the defined system boundaries – iron-ore miners from Brazil, manufacturers from Germany and transportation workers from both countries for the carbon steel frame; bauxite miners from China, manufacturers from Taiwan, and transportation workers from both countries for the aluminium frame; and miners from the Russian federation, manufacturers from Germany, and transportation workers from both countries for the titanium frame.
Based on the fair wage method introduced by Neugebauer et al. (Reference Neugebauer, Emara, Hellerström and Finkbeiner2015), FWPs are calculated for all listed groups of workers and processes. The data covering the supply chain are taken from secondary data sources.Footnote 10 The achieved results are shown in Figure 8.
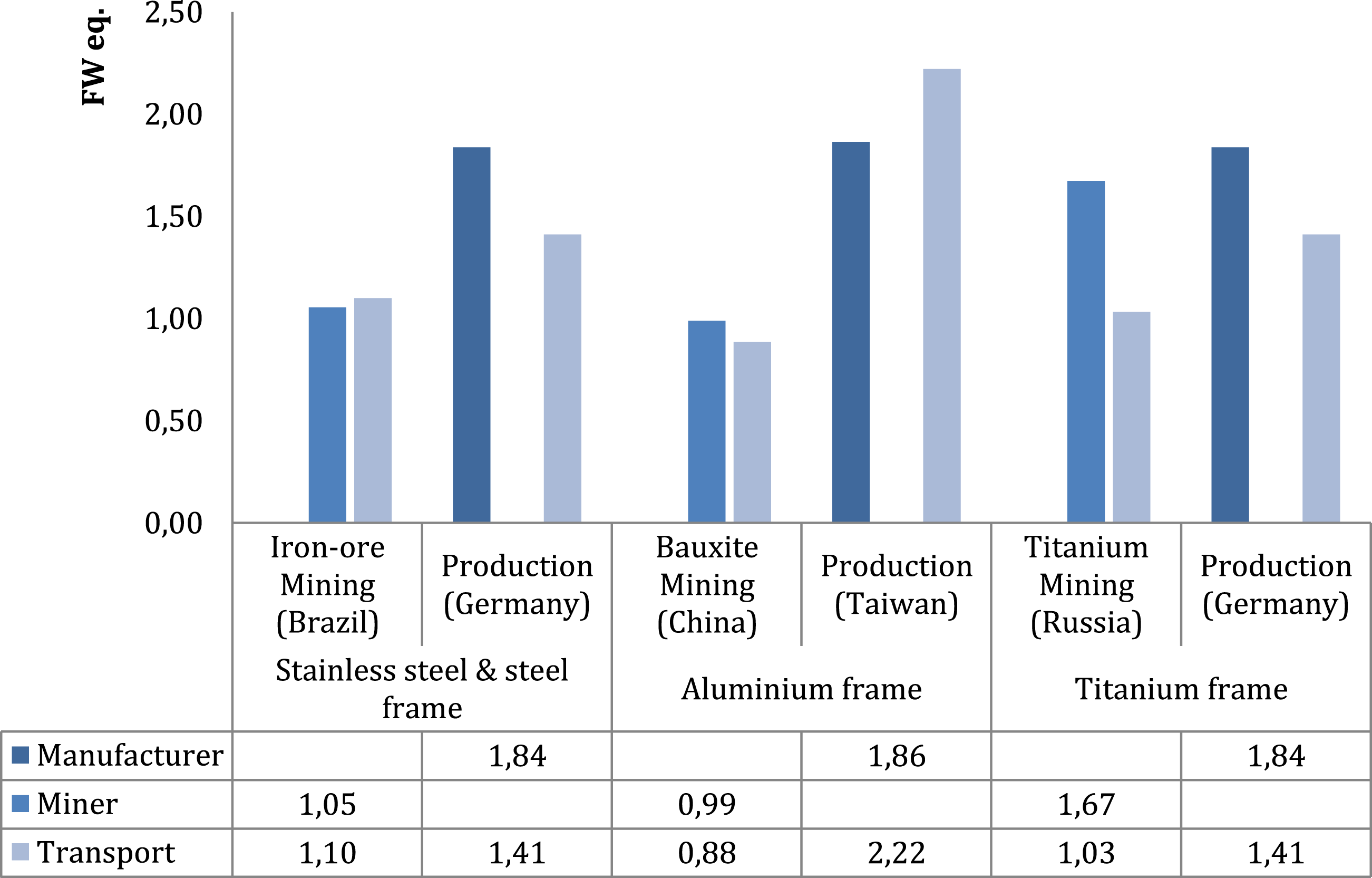
Figure 8. Fair wage potentials for the tricycle frames considering the different production locations.
Figure 8 shows that no negative social impacts result for the German and Taiwanese workers, as the values are
$\gg 1$
. The same accounts for the Russian miners. However, the FWPs calculated for the Chinese transport workers result in values
${<}1$
. Thus, negative impacts for the workers and their families can be expected.
Simplified economic life cycle assessment results
For the economic dimension, the study considers the value added by the frame producer, by comparing the different frame materials. Three categories are taken into account – material costs, operating costs by means of labour costs and the income achieved through sale. The included cost data are mainly based on assumptions due to the early stage in the development process. By this means, the labour costs can be estimated through typical hourly wagesFootnote 11 and process time.Footnote 12 Footnote 13 Profit margins for retailers are based on the current bike and pedelec market situation. A study performed by a German bank (ifo Institut 2013) concludes that the overall margin comes to 39.5%. The EHI Retail Institute,Footnote 14 in its statistical report, mentions 36% average benefits. In the case study, the more conservative percentage is included for calculating the value added.
The value added is then calculated by subtracting the costs per functional unit from the purchase prices achieved. The results can be taken from Figure 9.
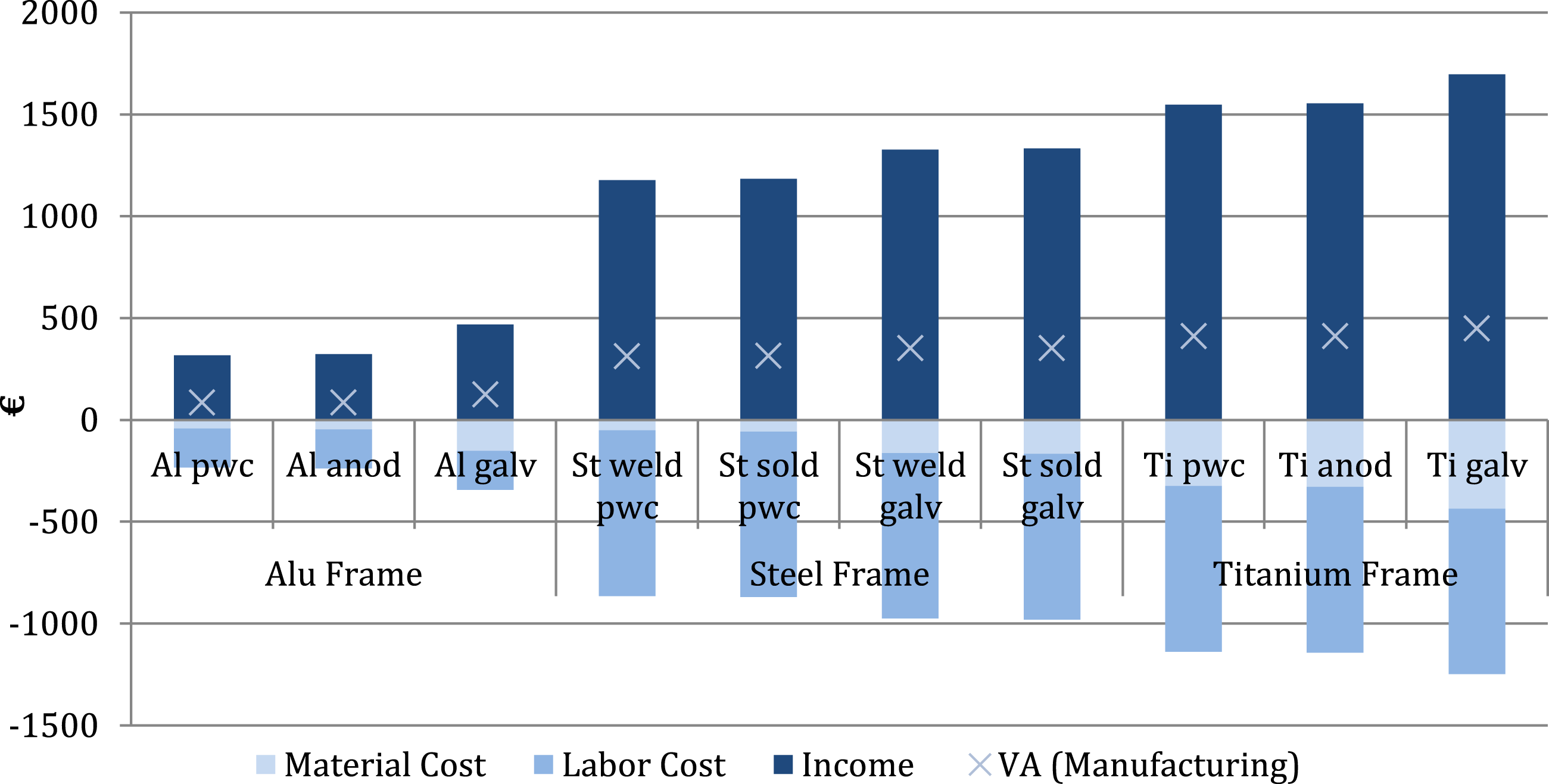
Figure 9. Value added by the different frames, considering the manufacturing sites in Taiwan and Germany.
Figure 9 shows that the value added by the titanium frame is higher than that of the steel and aluminium frames. The material cost in each case only contributes a small share to the overall costs, while the labour costs – especially for the steel and titanium frames – dominate the cost side.
Interpretation of the sustainability footprint results
While the steel and titanium frames are comparable from the social and economic dimensions, the steel frame outperforms the other two frame materials from an environmental point of view, by means of global warming potential. In addition to the outstanding environmental performance, steel provides further benefits in terms of manufacturability. Therefore, steel is chosen as a frame material for the further development of the new tricycle concept. However, it may have some shortcomings in terms of weight, which can increase the energy consumption during use phase and can also reduce the cycling comfort. Further investigations are required in this context.
5.3 Project outcome: The Smart Urban Wheeler Prototype
The SUW is the result of the considerations given in the last sections to develop a more sustainable mobility product. The prototype is now introduced, by specifying how the requirements formulated in Table 2 can be addressed.
5.3.1 Ergonomics/usability
Addressing the ergonomics and usability of the SUW, the distance between bottom bracket and seat (a), the handlebar position (b) and the backrest inclination (
$\unicode[STIX]{x1D6FC}$
) can be adjusted without stages, as shown in Figure 10. Furthermore, an ergonomic seat – in combination with a headrest and a fully dampened frame – is used.
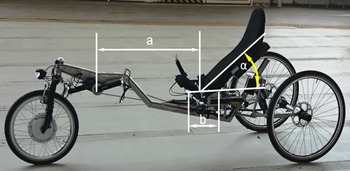
Figure 10. Configuration of the seating arrangement.
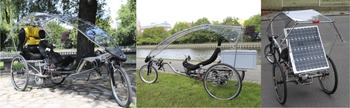
Figure 11. Rack configurations of the SUW prototype.
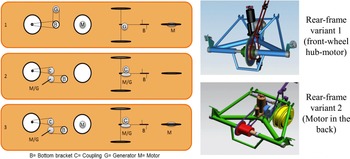
Figure 12. Achievable motor configurations.
Through the integration of a modular rack, which allows the attachment of a child restraint, a luggage box, or a solar panel (see Figure 11), the functionality of the SUW can be enhanced compared to typical delta tricycles. In addition, rain protection is ensured with a newly designed heat-resistant and transparent dome concept.
Modularity
In addition to the modular rack, multiple drivetrain variants are realized with the SUW. While Section 5.2.1 presents the chosen concept for a motor in the rear part of the SUW (see Configuration 2, Figure 12), another configuration with a front motor (see configuration 1, Figure 12) is added. Since most of the weight is situated in the rear part of the SUW, less traction can be achieved by a front motor, leading to less driving comfort. Nevertheless, the front motor configuration is less costly, since the entire design is less complicated. Therefore, people with lower incomes can be approached with this solution as an additional target group. The third configuration shown in Figure 12 is a combination of Configurations 2 and 1, with two hub motors – one in the front and one in the back. This configuration allows high travelling speed with 500 W and maximum speed of 45 km/h. In this case, German law requires a specific driver’s licence and insurance. All three drive configurations can be realized with the SUW.
User Interface
For the user interface of the SUW, a first interface prototype is implemented, based on a smartphone app. Therewith, a visualization of energy resources (solar energy, recuperation of braking energy, charging-while-standing, muscle strength, battery capacity) is possible in order to increase the awareness about driving-related energy production and consumption. In addition, tracking of the driving behaviour is possible. Concepts for intelligent training programmes or for automated prediction of optimal maintenance cycles – in order to increase the lifespan of the SUW – are future concerns and are to be implemented in the second project phase.
Energy concept
Measurements were conducted to evaluate the effectiveness of the implemented improvements of the energy concept. The solar panel mounted on the SUW rack was tested in parking position on three different days with varying cloud coverage. Cloud coverage is measured in Okta, ranging from 0 (clear sky) to 8 (completely clouded sky). The average cloud coverage in Germany from April to September 2016 was 4.74 Okta. The test condition which comes closest to this value is 5.7 Okta and is therefore taken as a reference. The generated energy was measured for an exposure time of 7.5 hours. In this period of time, 95.8 Wh/7.9 Ah could be generated. This amount of energy is sufficient to charge about 30% of the overall battery capacity of 324 Wh. Given a scenario of 103 days of pedelec usage per year with an exposure time of 7.5 hours per day of commuting, 68 kWh can be generated (for a usage period of 7 years). By assuming an average pedelec energy consumption of 1 kWh/100 km (German Federal Environment Agency 2014) and a daily travel distance of 25 km, around 37% of pedelec life cycle energy consumption can be generated by the solar panel. Nevertheless, this value is only possible if the solar panel is mounted on the rack, thus preventing the use of a luggage container or child restraint.
The ‘charging-while-standing’ function of the SUW was measured in five tests for three different gears (low, medium and high). The most effective setup was at an average engine speed of 48 RPM on medium gear. On average, 1.72 Wh could be generated for two minutes of pedalling. Hence, for generating 1 kWh, 19.3 h of pedalling is necessary. The recuperation of braking energy was tested in 12 trials on a flat street for a distance of 120 metres. The generated Wh were only measured for braking in the last part of the test distance. The highest amount of recuperated energy was 0.08 Wh for 23 seconds of braking for an average motor speed of 37 RPM. While the solar panel proved to be successful for reducing fossil fuel consumption, the added value of recuperation and ‘charging-while-standing’ need to be discussed.
Material
Following on the specifications of Table 2, two requirements should be considered in regard to materials – sustainability and light weight. While the chosen steel frame fulfils the first requirement, it may have shortcomings in regard to the second one. Steel is heavier than the other materials considered – aluminium and titanium. Whether – and to what extent – the potential energy losses during use phase outweigh the emission savings during production will be part of further research. However, considering the weight of the frame compared to the total weight of the SUW (approx. 63 kg in total), other parts may be more promising than the frame in terms of light weight.
6 Discussion of case study results
The results of the introduced design project are now reflected upon, to answer the two research questions raised in Section 1. This section is divided into two sections: focusing the SUW concept itself and the corresponding design process.
6.1 Discussion of the Smart Urban Wheeler concept
The following considerations follow the structure of the underlying framework for sustainability improvement, introduced in Figure 1.
Sustainability improvement and challenges at the mobility system level
To assess whether the SUW can be a meaningful contribution for increasing sustainability at the system level is a critical but complex task, as the system is dependent on multiple variables, such as policies (e.g., subsidy for electric cars) or potential lock-in effects, through established means of transportation (e.g., company-owned cars). However, as the SUW is designed to convince potential customers to use a pedelec instead of a car, the market potential of the idea might be a first hint. For testing whether customers appreciate the SUW for the mentioned purpose, the prototype was introduced to the general public at a TU Berlin science fair in May 2016. Discussions with 18 different people yielded qualitative feedback on the concept. In general, the potential of the SUW to substitute small passenger cars was recognized by almost everyone. Furthermore, the SUW features for energy recovery and modularity were perceived as beneficial. A common criticism of the concept was a lack of heating options for the convenient operation of the SUW in winter or bad weather situations. To address this challenge, the utilization of infrared heating devices could be a solution, since they do not rely on a closed driver’s cabin to keep the driver warm. A negative effect is the high energy consumption of electrical heating. A closed-roof variant would be more efficient, but would also be less beneficial in summertime. A detachable and foldable roof concept may be a solution for this purpose. Such a solution would also come in handy to improve the ergonomics of entering the SUW, particularly for taller people. Another concern of the interviewed people was the high cost of the SUW. In the dialogues, an overall purchase price of €6000–8000 was estimated assuming serial production. People were generally hesitant to pay such a high amount of money since, they can acquire a used passenger car for that price. While cost is not an explicit requirement for the first SUW prototype, further development is necessary to identify cost reduction potential.
Sustainability improvement and challenges on mobility solution level
Another challenge for the SUW to have a long-lasting impact is a sustainable business model. Due to the high purchase price, alternative models to privately owned vehicles – such as product service systems – may be taken into account. Sharing schemes reduce the number of manufactured vehicles and also save on urban space. Public bicycle sharing systems already exist in many metropolitan areas (e.g., Berlin). However, as the value of an SUW is much higher than a standard bicycle, public sharing schemes provide higher risk of theft and damages to the vehicle, particularly for free-floating sharing systems. Another argument against a public sharing scheme limited to the city is the high competition to already existing car and bicycle sharing systems. As an alternative, private sharing systems – for example, organized by firms and organizations – show great implementation potentials. Pedelecs from company-owned pools may be utilized for daily commuting and could therefore contribute to the substitution of a car used in the household, even outside Berlin. Companies can profit by a green image to the outside and health benefits towards their employees. A more detailed concept of such a product service system for the SUW can be found in Barquet et al. (Reference Barquet, Seidel, Buchert, Galeitzke, Neugebauer, Oertwig, Rozenfeld and Seliger2016). In this study, a complete business model for a private sharing system at the Technical University of Berlin is evaluated. While the primary use case of the SUW is seen in commuting, the field of application could also be extended to delivery or tourism. However, further studies are necessary for this purpose.
Sustainability improvement and challenges on mobility product level
Concerning the SUW, sustainability has been evaluated for the whole product (by utilizing DSS guidelines) at the assembly level (SUW architecture and drive concept) and the part level (SUW frame). The following points remain unsolved and may be addressed in future work:
-
(i) Until now, respective sustainability assessment studies have primarily been conducted for self-developed parts. To verify that the SUW is equipped with the best possible configuration of components in terms of sustainability, all sourced parts would need to be evaluated against comparable alternatives available on the market in further studies.
-
(ii) The SUW weight is very high at the moment. Further weight improvements may be possible by reducing the safety factors of the components.
-
(iii) Measurements of the energy concepts show that the solar panel is quite effective in reducing use-related emissions of pedelecs. It should be investigated whether the solar panel can be integrated into the dome instead of the rack to guarantee constant energy supply.
-
(iv) Measurements of recuperation and ‘charging-while-standing’ prove that the amount of energy harnessed is low. For proper utilization of ‘charging-while-standing’, further application cases of the SUW need to be evaluated (e.g. as a sport equipment). Recuperation can be more useful in hilly environments. Urban areas may therefore not be the most suitable application field in most cases.
6.2 Reflection on the design process and its influence on product sustainability
Complementary to the developed mobility solution, the design process is observed and analysed in terms of obstacles and success factors for SPD.
Utilized SPD methods
Three different methods have been utilized to guide the development process of the SUW towards a more sustainable alternative in comparison to other transport alternatives. In this context, the suitability of the approaches to the framework of the study (see Figure 1) is evaluated. As a basic requirement for their selection, all three methods provide a focus on life cycle thinking and all three sustainability dimensions (while implications on the social perspective are less represented as they are still subject of research). However, like most methods in the field of SPD or Ecodesign, the approaches are limited to considerations at the product level. Hence, an overarching perspective on the business model of the company and the system in which the product is utilized is not supported. A method that offers such a holistic approach could not be identified in previous literature research. At the product level, the design team could generally make use of the selected methods, as they provide helpful pivots for improvement (DSS), criteria for comparison (ProdSI) and quantitative reference (LCSA). In particular, the combination of quantitative and qualitative methods is seen as helpful for the design team for analysing the product from different perspectives.
One challenge faced by the design team is the appropriate selection of criteria for sustainability evaluation. Both methods – LCSA and ProdSI – have a large number of criteria that cannot be fully considered in the design phase due to time constraints of the project. Hence, the set of criteria needs to be simplified. While Neugebauer et al. (Reference Neugebauer, Martinez-Blanco, Scheumann and Finkbeiner2015) provide an approach for LCSA indicator selection, application of ProdSI requires the classification of 47 indicators into six groups. Another problem in this context is the limited amount of information for evaluating the indicators. In particular, towards the beginning of the project, it would have been helpful to obtain quantitative reference for comparing different technologies and product concepts (e.g., the driving concept or the pedelec architecture). Hence, approximations were used (e.g., efficiency of a driving concept), which may be sufficient for the initial estimation of performance but can lead to a wrong decision in the trade-off analysis. In this context, the design team also faced difficulties in including qualitative factors in the (ProdSI) analysis. Trade-off analysis involving qualitative factors (e.g., a surplus on safety vs emission reduction) only provides a vague basis for decision-making and leaves room for interpretation and subjectivity.
Decision-making process
As the first challenge concerning decision-making in the project, the formulation of the project aim concerning the debate of absolute versus relative sustainability needs to be discussed. As there are no scientifically valid boundaries available to define whether a product is sustainable or not, relative sustainability is used as a reference. Relative sustainability builds on a relative comparison of two objects with similar functions and means that a solution is more sustainable than another one if at least one of considered sustainability indicators performs better while no other criterion is compromised. While this approach has a certain appeal in its simplicity, it leads to some problems in practice. A major criticism in this context is the unrealistic assumption that all relevant indicators can be considered, as this often leads to too much effort in day-to-day business. Furthermore, there is a methodological problem connected to the comparison itself, which requires functional equivalence. In the SUW project, an existing pedelec of the type Hase Klimax2k is used as first reference to monitor the effects of improvement. However, as the SUW has a multitude of additional functions, the basis for comparison is lost.
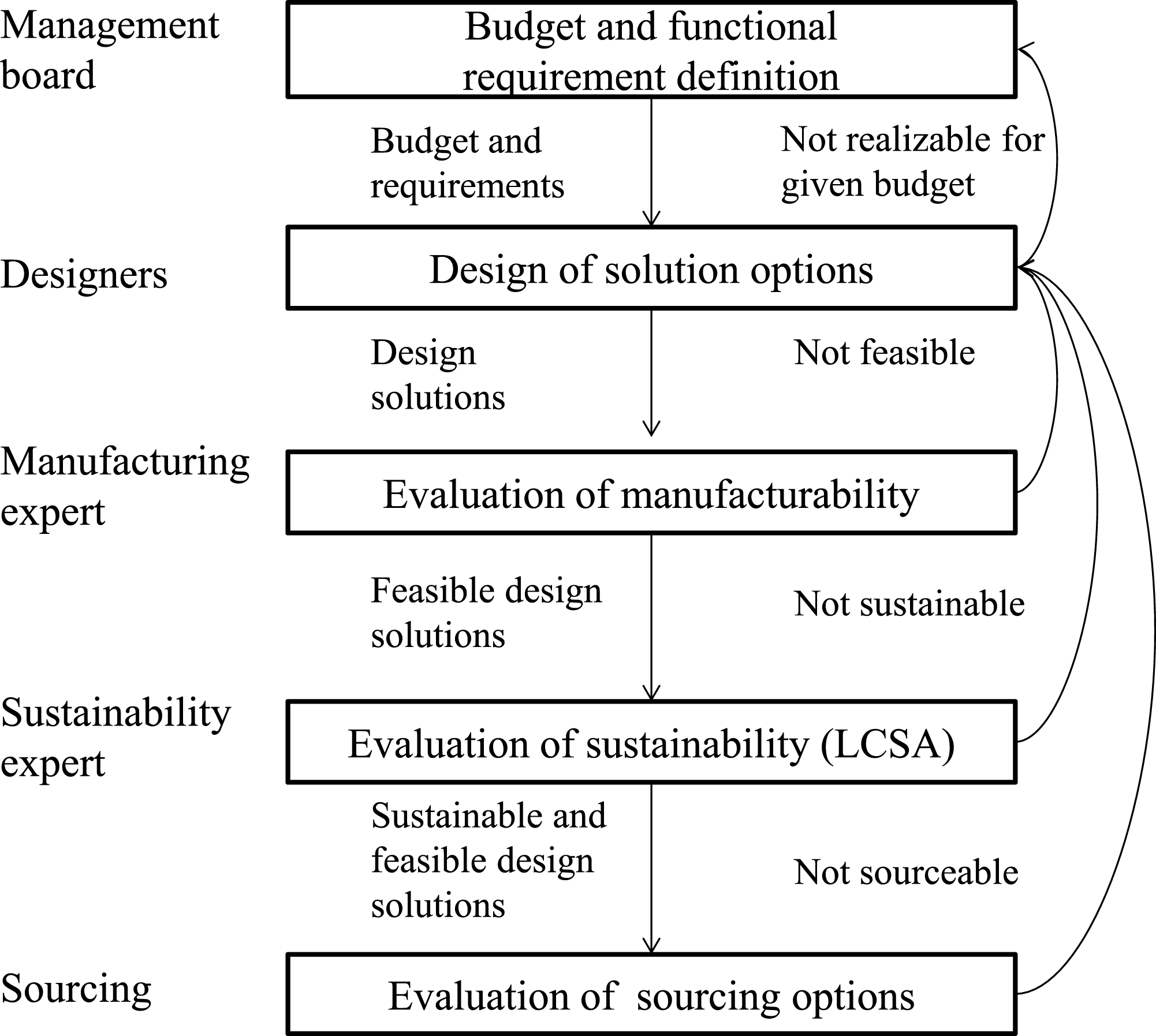
Figure 13. Decision-making process in the project.
Another challenge concerning decision-making in the project is caused by the process of making decisions in the project team. As the project follows well-established design approaches like Simultaneous/Concurrent engineering or Integrated Product Development, decisions in the project were made by an interdisciplinary team consisting of designers, project managers, a manufacturing engineer, and a sustainability expert, by applying the LCSA method. The regular meetings of the people behind these roles enabled a more informed debate about the consequences of design decisions and led to a broad spectrum of fruitful ideas. However, in some cases, decision-making involving the additional perspective of sustainability proved an obstacle to project progress and success. The decision-making process (see also Figure 13) started with the definition of design options based on a given budget and functional requirements of the management board. For example, the selection of materials suitable for the SUW dome and frame required the definition of two or three material options suitable for achieving technical requirements (forces, temperature etc.). This step already involves a large amount of effort due to search for material specifications and simulation of product behaviour. After this, the options were evaluated concerning manufacturability and a revision of design options was necessary in some cases. In the next step, all manufacturable alternatives were presented to the sustainability expert for conducting LCSA. Performing an LCSA also requires much work and time for the required analysis. Hence, a long lead time (2 weeks to 1 month) had to be provided. Through LCSA analysis, material options were ranked. In the case of the SUW frame, the material ‘Advanced High Strength Steel’ (AHSS) was identified as the most beneficial option. Despite good sustainability performance and manufacturability, it was not possible to acquire the material, since it is used primarily for car manufacturing and therefore only offered in very large batches to automobile OEMs. Similar problems were experienced for sourcing the material for the dome. Hence, there were long iteration cycles between design, manufacturing, sustainability assessment, and sourcing, which led to time pressure for achieving project milestones. The time pressure in turn caused a constant struggle between achieving technical functionality for milestone reviews and proper optimization of sustainability performance. One example in this context is the lack of time for proper structural analysis of the pedelec frame in terms of material savings and weight reduction. Hence, many frame parts are designed with a higher safety factor than required.
While in the beginning, the project was primarily focused on identifying and prioritizing relevant decision criteria for major concept decisions, the focus of the project gradually shifted towards achieving the goals in a certain timeframe by the project end.
7 Conclusion
While there is some consensus in the scientific community on the basic theory behind SPD (see Section 2), there is still no agreed standard available to help companies in implementing these principles in their products. Since the number of available SPD methods is already quite high, it may be beneficial to concentrate on improving the most promising candidates instead of constantly developing new methods. Further application studies on the existing SPD approaches are necessary to see how these approaches can be applied to real projects, in order to identify potential for improvement. On the concrete example of the SUW development project, three SPD methods were tested. This combination of qualitative and quantitative approaches was seen as a helpful basis for communication within the project team and to gather an improved understanding of the product. However, it was also recognized that applying sustainability methods does not automatically lead to a more sustainable product, as design decisions in a real project situation depend on project constraints imposed by budget, time, and individual preferences/motivations of the involved stakeholders. One of the difficulties faced by the project team in this context is the large variety of different criteria for SPD analysis and synthesis on product level. Due to limited time and resources in the project, a deep analysis of every criterion was not possible. The large number of design criteria also increased the necessity of information exchange between project stakeholders (e.g., for supplying product information for LCSA) and delayed important decision-making processes.
Despite the academic setup of the case study, it can be argued that pressure concerning time and resources in design projects is also transferable to industrial design projects. To improve the situation, the following recommendations are made for streamlining the utilization of existing SPD methods:
-
(i) To simplify the selection of most relevant sustainability measures, a classification scheme is necessary which makes transparent the suitability of indicators for different product categories. One step in this direction could be the approach of Environmental Product Declaration (EPD), which defines specific product category rules for environmental assessment. Hence, it should be evaluated how this approach can be extended to the framework of sustainability.
-
(ii) Complementary, simpler screening approaches for SPD are required to provide information on all three sustainability dimensions without extensive information and time demand for analysis.
-
(iii) To further decrease the effort needed for the acquisition of sustainability information – in particular for early phases of the design process – appropriate knowledge management is necessary (e.g. by feeding back information available from predecessor products). Appropriate processes, IT tools, and central data repositories can also help in speeding up the decision-making process.
Concerning the case study approach itself, the following insights are seen as valuable for further research on SPD methods:
-
(i) Using a framework – such as the one presented in Figure 1 – helps to understand which elements can be considered for developing sustainable products. It also provided a beneficial reference to evaluate the SPD methods utilized in this case study. Hence, this approach can be used as a blueprint for further method application tests.
-
(ii) A method that provides an integrated view on the product, corresponding services, and business models, as well as the overarching system in which the product is embedded, is currently not available as these relations are very product- and context-specific. Furthermore, methods that consider all three sustainability dimensions are scarce.