Altered volcanic ash may provide insights into the dynamics of trace-element depletion and enrichment during the low-temperature, post-emplacement alteration of primary igneous lithologies due to the increased solubility of trace elements under such conditions (McHenry, Reference McHenry2009; Hong et al., Reference Hong, Algeo, Fang, Zhao, Ji and Yin2019; Namayandeh et al., Reference Namayandeh, Modabberi and López-Galindo2020). Distal volcanic tephra, and ash in particular, consist largely of metastable volcanic glass, which is prone to deuteric alteration in aqueous environments (Christidis & Huff, Reference Christidis and Huff2009; Huff, Reference Huff2016). A comparison between fresh tephra and its alteration derivatives therefore offers an excellent medium to investigate trace-element post-emplacement trajectories (Summa & Verosub, Reference Summa and Verosub1992; McHenry, Reference McHenry2009). Several studies have utilized this approach to demonstrate element behaviour during glass argillization and zeolitization in marine and freshwater environments (Christidis, Reference Christidis1998; McHenry, Reference McHenry2009; Kiipli et al., Reference Kiipli, Hints, Kallaste, Verš and Voolma2017; Hong et al., Reference Hong, Algeo, Fang, Zhao, Ji and Yin2019). These studies were focused on residual elements to infer material provenance and the variations in trace element contents were used to reconstruct the physicochemical character of the depositional environment. The aforementioned research relied on sampling from various localities (i.e. fresh vs altered) followed by whole-rock geochemical analyses of the collected samples. This approach required relatively large amounts of analysed material and was prone to possible contamination by non-authigenic detritus. The present study attempts to reconstruct trace-element mobility patterns by investigating the spatially associated fresh volcanic material and the clay minerals it produces. Two samples of partially altered Miocene volcanic ash from the Dinaride Lake System (DLS; Fig. 1; Krstić et al., Reference Krstić, Dumurdžanov, Olujić, Vujnović and Janković-Golubović2001; de Leeuw et al., Reference de Leeuw, Mandic, Krijgsman, Kuiper and Hrvatović2011; Mandic et al., Reference Mandic, de Leeuw, Vuković, Krijgsman, Harzhauser and Kuiper2011; Šegvić et al., Reference Šegvić, Mileusnić, Aljinović, Vranjković, Mandic and Pavelić2014; Badurina et al., Reference Badurina, Šegvić, Mandic and Slovenec2021) were selected for laser ablation inductively coupled plasma mass spectrometry (LA-ICP-MS) in situ analyses of glass shards and their respective clay (<2 μm) separates.
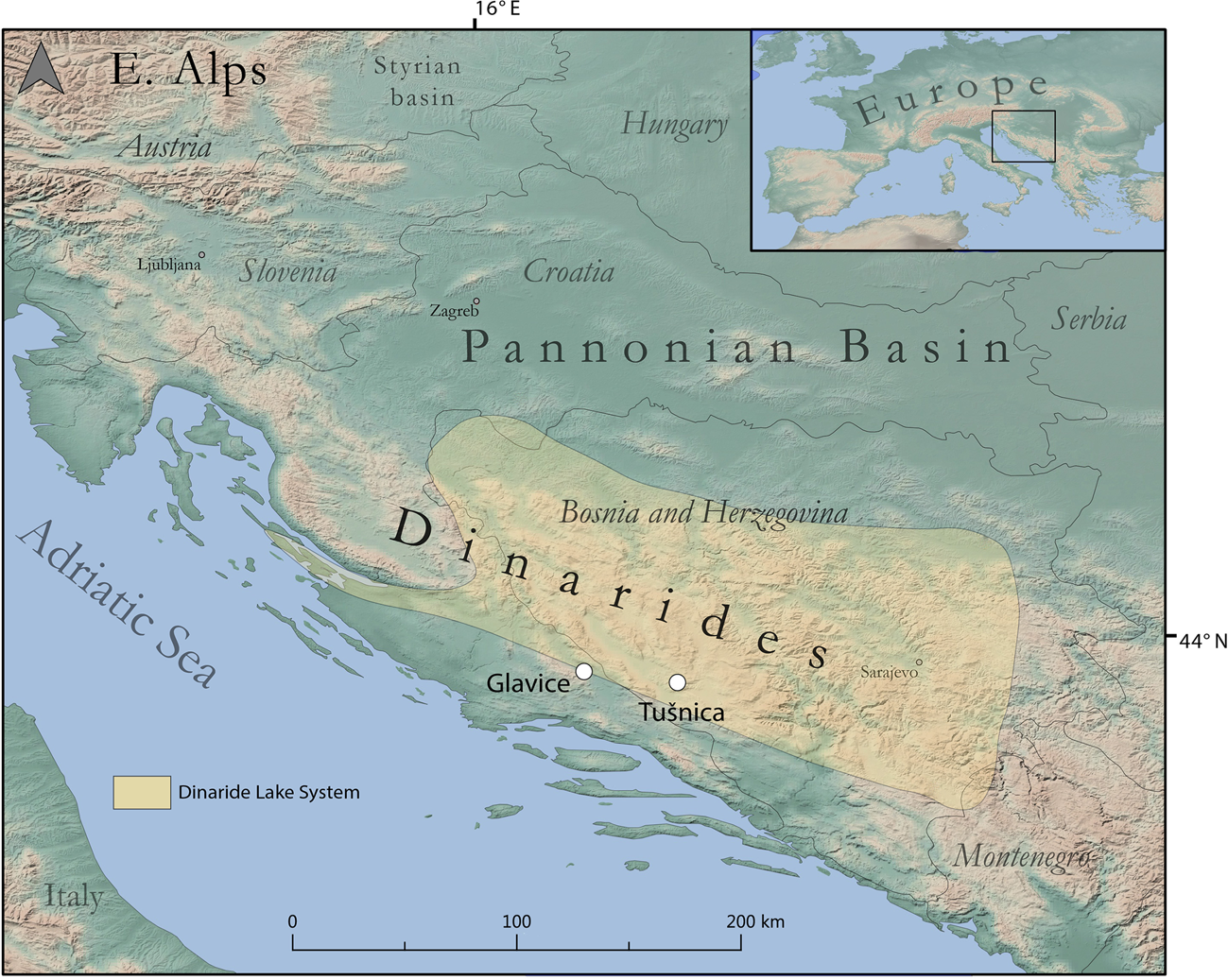
Fig. 1. Geographical map of the sampling localities (modified after Badurina et al., Reference Badurina, Šegvić, Mandic and Slovenec2021).
This enabled direct assessment of trace-element content in spatially related parent/daughter materials based on very-low-volume analyses of glass shards and tiny amounts of clay separate (~50 mg). X-ray diffraction (XRD), Fourier-transform infrared (FTIR) spectroscopy and scanning electron microscopy with energy-dispersive X-ray spectroscopy (SEM-EDS) observations were used to characterize the mineralogy and geochemistry of the clay minerals and to identify the possible presence of detrital components.
Materials and methods
Material for this study was recovered from the central DLS localities of Glavice and Tušnica (Croatia and Bosnia and Herzegovina, respectively; Fig. 1; de Leeuw et al., Reference de Leeuw, Mandic, Krijgsman, Kuiper and Hrvatović2011; Šegvić et al., Reference Šegvić, Mileusnić, Aljinović, Vranjković, Mandic and Pavelić2014; Badurina et al., Reference Badurina, Šegvić, Mandic and Slovenec2021). Bulk and clay-fraction XRD analyses were carried out at the Geosciences Clay Laboratory of Texas Tech University (TTU; analytical details by Šegvić et al., Reference Šegvić, Zanoni and Moscariello2020). Measurements were performed using a step scan in the Bragg–Brentano geometry with Cu-Kα radiation (40 kV and 40 mA) and a counting time of 3.5 s per 0.019°2θ from 3.8 to 70°2θ for bulk fractions, which was modified to 2.3 s per step count from 3.8 to 33°2θ for clay-fraction measurements. The infrared-attenuated total reflectance (IR-ATR) spectra at ambient conditions were collected using a Perkin Elmer Frontier MIR spectrometer, installed in the same laboratory, in a range of 800–1100 cm–1 with a spectral resolution of 2 cm–1. The SEM-EDS analyses were performed at the Microscopy Center of the College of Arts and Sciences of TTU as described by Badurina et al. (Reference Badurina, Šegvić, Mandic and Slovenec2021). Tuff sections containing glass shards were prepared in the form of thick (~100 μm) sections and analysed using LA-ICP-MS at the TTU GeoAnalytical Laboratory (analytical details in Šegvić et al., Reference Šegvić, Zanoni, Bozkaya, Sweet, Barnes, Boulesteix and Solé2021). Si abundances determined using EDS were utilized as internal reduction standards. The clay fractions from the studied samples were separated using centrifugation (Šegvić et al., Reference Šegvić, Zanoni, Bozkaya, Sweet, Barnes, Boulesteix and Solé2021) and then investigated using a LA-ICP-MS line raster analysis (Table S1), which has been proven to be suitable for the study of an inherently heterogeneous clay material (Vannoorenberghe et al., Reference Vannoorenberghe, Acker, Belza, Teetaert, Crombé and Vanhaecke2020). The time-resolved LA-ICP-MS signal was inspected for possible contamination. The matrix-appropriate standards Jsd-1 and USGS BHVO-2 G (Jochum et al., Reference Jochum, Willbold, Raczek, Stoll and Herwig2005; Nath et al., Reference Nath, Makishima, Noordmann, Tanaka and Nakamura2009) served as measures of the method's precision and accuracy (Table S1).
Results and discussion
Mixed-layer illite-smectite (Ilt-Sme) accompanied by minor amounts of illite, kaolinite, tridymite, quartz, feldspar and gypsum make up the bulk mineralogy of the analysed tephra (Fig. 2a,b). The prominent 15–35°2θ hump in the XRD traces (Fig. 2a,b) is attributed to the presence of volcanic glass and possibly amorphous silica (i.e. opal-A). The XRD traces of the bulk samples and the clay fractions of both samples show a predominance of highly crystalline Ilt-Sme. This is somewhat less pronounced in the case of the Glavice sample, which encompasses multiple Ilt-Sme generations of lower crystallinity. The relative positions of the Ilt-Sme 002/003 peaks (15.78 vs 15.69°2θ) combined with the 001/002 and 002/003 Δ°2θ values (5.33 vs 5.31Δ°2θ; Moore & Reynolds, Reference Moore and Reynolds1997) suggest a Sme-rich (i.e. >90%) Ilt-Sme in both clay fractions, with the Glavice sample probably having a slightly greater Sme content.
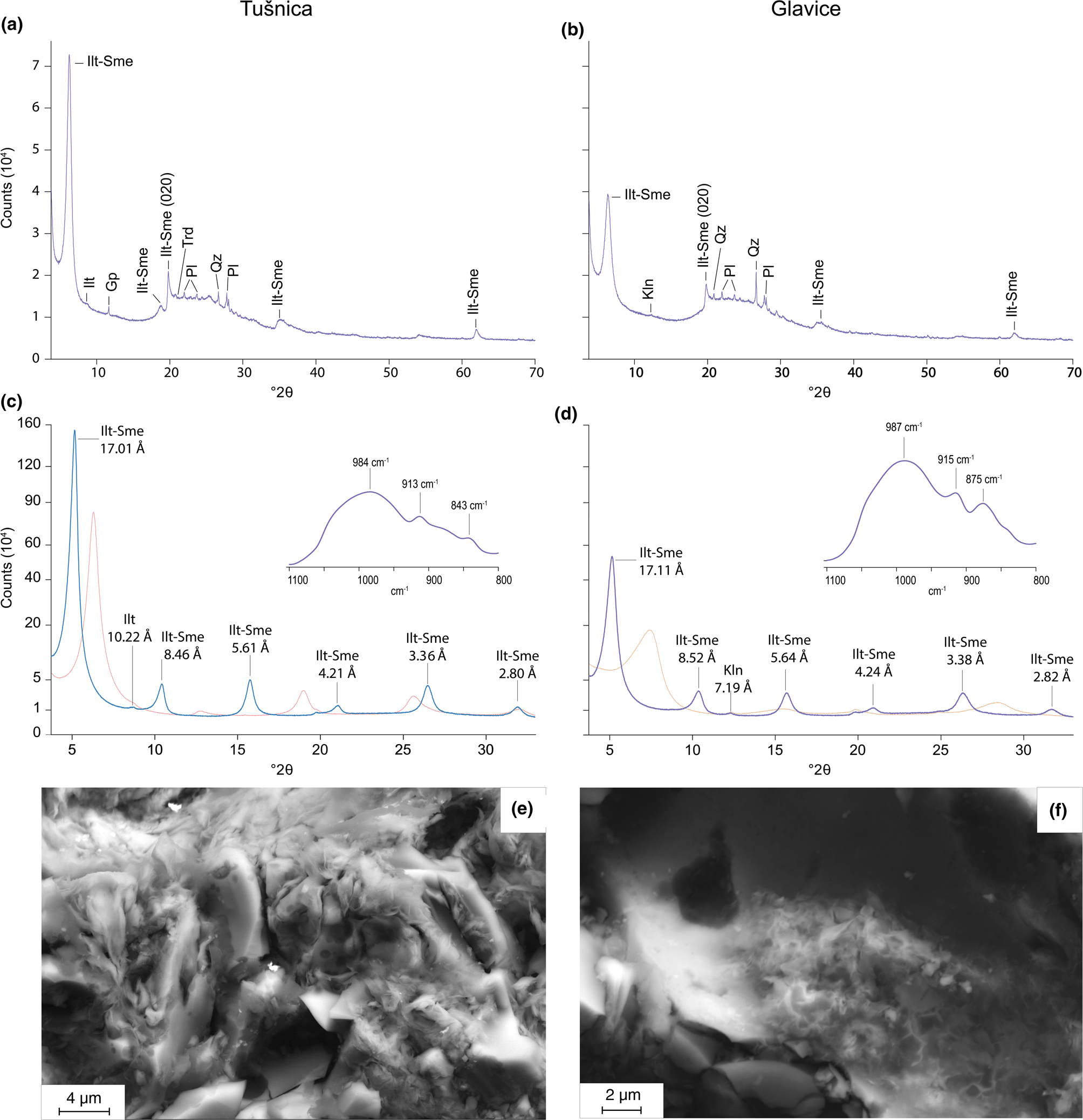
Fig. 2. XRD traces of (a,b) bulk and (c,d) clay fractions (<2 μm). The insets indicate FTIR spectra of the clay fractions. The red and blue lines in the XRD traces of the clay fractions correspond to air-dried and ethylene glycol-solvated samples, respectively. The y-axis values were square-root transformed to assist with the correlation. (e,f) SEM images of the Tušnica and Glavice samples, respectively. Ilt-Sme = illite-smectite; Ilt = illite; Kln = kaolinite; Trd = tridymite; Qz = quartz; Gp = gypsum; Pl = plagioclase.
The IR-ATR spectra in both samples reveal Si–O and Al–Al–OH bands at ~990 and 914 cm–1, respectively (Fig. 2c,d). The Tušnica sample features an 843 cm–1 Al–Mg–OH band, while the Glavice sample displays an Al–Fe–OH band at 875 cm–1 (Fig. 2c,d; Madejová et al., Reference Madejová, Gates, Petit, Gates, Kloprogge, Madejová and Bergaya2017).
The SEM-EDS analyses reveal glass shards of essentially constant chemical composition that seem to be coated with Ilt-Sme of variable morphology (Table 1). Ilt-Sme outgrowths of similar phase chemistry emerge readily from the glassy substrate (Fig. 2e).
Table 1. EDS and LA-ICP-MS analyses of the studied tuff. Major oxides and trace elements are expressed in wt.% and ppm, respectively.

bdl = below detection limit.
In the case of the Tušnica clays, the Ilt-Sme crystals are up to ~0.3–2.0 μm long (Fig. 2e & Table 1). Conversely, the size of Ilt-Sme in the Glavice clays is smaller, being within the sub-micron range. These clays form aggregates with a typical honeycomb texture (Christidis, Reference Christidis and Christidis2010) found as glass vesicle infills (Fig. 2f). In comparison with volcanic glass, the observed clay minerals are depleted in SiO2 and alkalis while being enriched in all other oxides. The FeO and TiO2 in the clay minerals may be related to the decomposition of igneous phases such as biotite, while the increased MgO and CaO are attributed to the availability of these cations in karstic lake waters (Sironić et al., Reference Sironić, Barešić, Horvatinčić, Brozinčević, Vurnek and Kapelj2017). With reference to the Tušnica clays, the SiO2/Al2O3 ratio of Ilt-Sme in the Glavice clays is greater (3.8 vs 3.5; Table 1), which is consistent with the XRD data. The K and Na contents of the Glavice clays are greater than those of the Tušnica clays (1.8 vs 0.8 wt.% and 1.0 vs 0.6 wt.%, respectively; Table 1), while the octahedral Mg content seems to be halved (Fig. 2c & Table 1).
Chondrite-normalized LA-ICP-MS analyses of the volcanic glass revealed an evolved igneous character (LREE enrichment (LaN/Ybn (Tušnica) = 3.5, LaN/Ybn (Glavice) = 8.6), Eu anomaly (Eu*(Tušnica) = 0.3, Eu*(Glavice) = 0.4; Fig. 3a,b & Table 1).
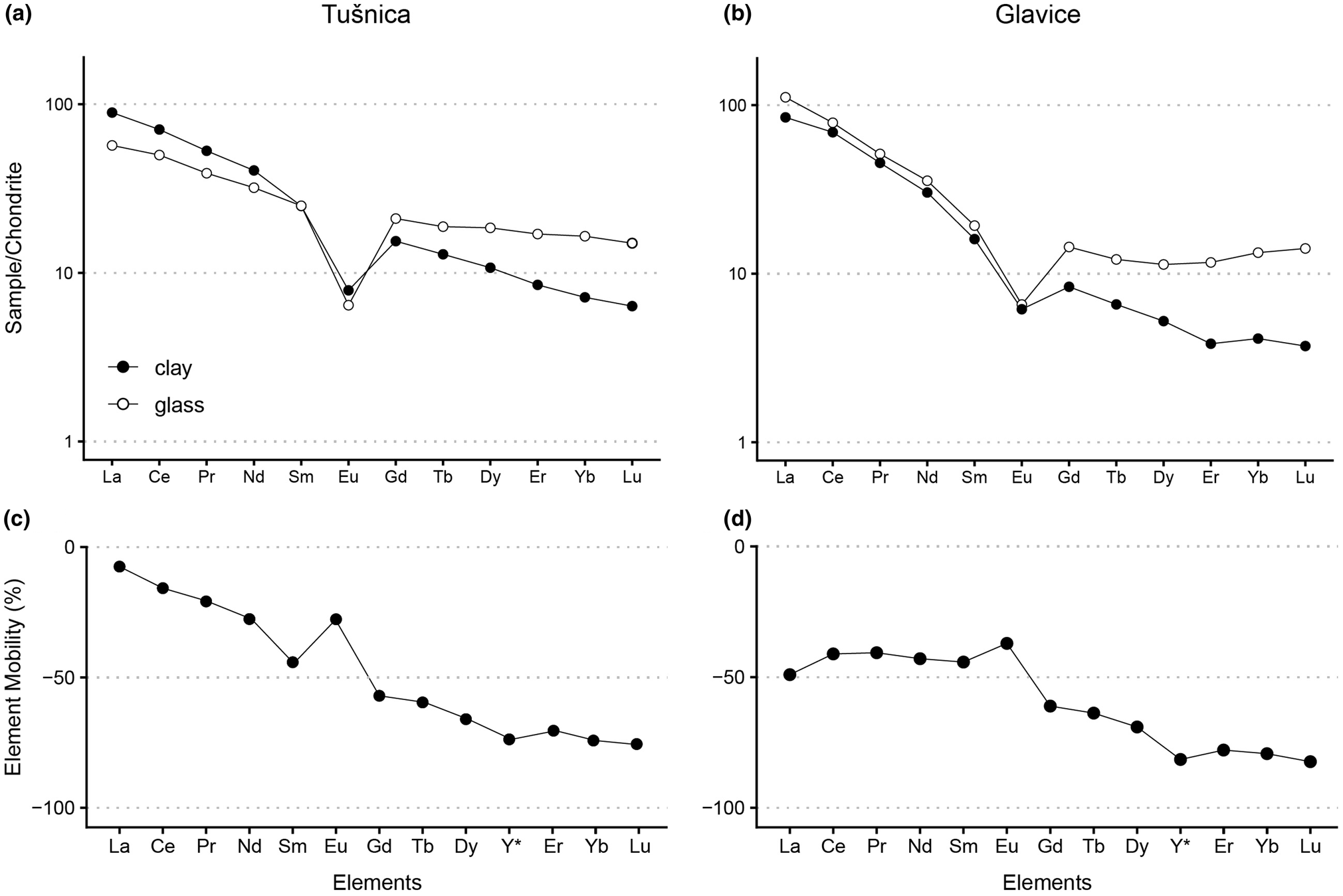
Fig. 3. (a,b) Chondrite-normalized plots (Boynton, Reference Boynton and Henderson1984) and (c,d) element mobility plots of the analysed samples.
The same normalization applied to the REE of the clay fractions resulted in greater LREE/HREE ratios (LaN/Ybn (Tušnica) = 12.8, Lan/Ybn (Glavice) = 20.5; Fig. 3a,b & Table 1) and Eu anomalies of reduced intensities (Eu*(Tušnica) = 0.4, Eu*(Glavice) = 0.5). To inspect trace-element mobility during the argillization of volcanic glass, we first normalized the clay and glass Y and REE contents to the respective Al2O3 concentrations of the samples. The two normalized samples were then compared against each other using the equation of Nesbitt (Reference Nesbitt1979) (Fig. 3c,d). Both samples feature similar rates of Y and HREE depletion (~70%) and positive Eu anomalies (Fig. 3c,d). The LREE depletion rate of the Glavice clays remains flat at ~40%, while in the Tušnica clays the LREE tends to deplete systematically (~7 to ~40% loss; Fig. 3d).
The high rates of Y and HREE depletion in the Ilt-Sme studied herein may be attributed to carbonate complexation of those elements and limited adsorption of these complexes on the clay mineral surfaces (Byrne & Kim, Reference Byrne and Kim1990; Sholkovitz et al., Reference Sholkovitz, Landing and Lewis1994). Various carbonate species presumably were abundant in karstic lakes such as the DLS (Cantrell & Byrne, Reference Cantrell and Byrne1987; Möller & Bau, Reference Möller and Bau1993; Christidis, Reference Christidis1998). In such environments, the REE distribution is controlled by sorption (Chen et al., Reference Chen, Algeo, Zhao, Chen, Cao, Zhang and Li2015), and a positive Eu anomaly as depicted in the mobility diagrams (Fig. 3c,d) commonly is related to plagioclase weathering (Weill & Drake, Reference Weill and Drake1973; Christidis, Reference Christidis1998). However, the DLS tephra is poor in plagioclase (Šegvić et al., Reference Šegvić, Mileusnić, Aljinović, Vranjković, Mandic and Pavelić2014); therefore, the Eu anomaly reflects a preferential mobilization of divalent Eu in anoxic porewaters rich in organic matter (Bau, Reference Bau1991; Hong et al., Reference Hong, Algeo, Fang, Zhao, Ji and Yin2019). The latter probably was derived from the neighbouring coal layer in the Tušnica clays (Badurina et al., Reference Badurina, Šegvić, Mandic and Slovenec2021). Particles of Ilt-Sme are dominated by their basal siloxane surfaces, which are the least reactive surfaces in clay minerals (Schoonheydt & Johnston, Reference Schoonheydt, Johnston, Bergaya and Lagaly2013). However, in the case of isomorphism and particularly octahedral substitution of Al by Mg, the charge deficit is delocalized over the surface oxygen atoms of siloxane planes (Sposito et al., Reference Sposito, Skipper, Sutton, Park, Soper and Greathouse1999). This represents a favourable adsorption domain for solvated cations such as LREE, which are suggested to vacate the glass in the form eight- or nine-fold hydrated outer-sphere complexes (Borst et al., Reference Borst, Smith, Finch, Estrade, Villanova-de-Benavent and Nason2020). A relatively great Mg content in the Tušnica clays, as is corroborated by the FTIR (Fig. 2c) and EDS spectra (Table 1), may therefore explain the pronounced LREE retention potential of these clays.
Conversely, the smaller particle size of the Glavice clays (Fig. 2f), which leads to a greater density of edge/defect charges, probably played a secondary role in LREE adsorption. Finally, the octahedral sheet of the Ilt-Sme in the Glavice clays seems to host Fe, which, in addition to the substitution of Mg by Al (Table 1), is further supported by the noticeable FTIR Al–Fe–OH band (inset in Fig. 2d). Under the oxidizing conditions that prevailed in Lake Sinj (Glavice locality; Vranjković, Reference Vranjković2011), structural Fe3+ did not contribute to permanent structural charge and therefore had no impact on the surface geochemistry of the Ilt-Sme.
Conclusions
This study has demonstrated that meaningful geochemical data can be collected from small volumes of a clay fraction (~50 mg) and individual glass shards to reconstruct the behaviour of trace elements during the argillization of pyroclastites in aqueous media. This enables analysis of spatially related parent and daughter materials, thus enabling the detection of possible (detrital) contamination. This approach is also useful for studying scarce materials from drill holes devoid of fresh equivalents. Subject to future investigation, this study has also shown that, in the case of 2:1 clay minerals (i.e. mixed-layer Ilt-Sme), it is their octahedral substitution that exercises primary control over the clay surface geochemistry. This gives rise to reasonably strong adsorption of the solvated complexes of trace elements. Our preliminary study focuses on the lacustrine environments of the DLS, where subtropical Miocene weathering of distal tephra produced clay assemblages with REE adsorption potential.
Supplementary material
To view supplementary material for this article, please visit https://doi.org/10.1180/clm.2022.12
Acknowledgements
The authors thank Kevin Werts for providing excellent LA-ICP-MS measurement conditions, Oleg Mandic for assisting with sample collection, James Browning for his kind assistance in thick-section preparation, three anonymous reviewers for their critical comments and constructive reviews and Asuman Günal Türkmenoğlu and George Christidis for their editorial assistance, which contributed significantly to the quality of this manuscript.
Financial support
This work was supported by the Summer Research Scholarship of Department of Geosciences and Geosciences Clay Laboratory of Texas Tech University.