Epidemiological and experimental studies have shown that malnutrition during critical periods of embryonic development or in infant diet after birth is an important predisposing factor for the development of obesity, diabetes, CVD and hypertension in adulthood(Reference Gluckman, Lillycrop and Vickers1–Reference Ozanne and Hales3). This phenomenon is referred to as ‘developmental programming’ or ‘developmental origins of health and disease’(Reference Gluckman and Hanson4). In humans, the risk of developing late-onset diseases is increased in infants born prematurely (at < 37 weeks of gestation), born from undernourished(Reference Barker, Osmond and Kajantie2) or overnourished mothers(Reference Cnattingius, Villamor and Lagerros5) or born with low body weight (weighing < 2500 g at birth)(Reference Barker, Osmond and Kajantie2). In the 1990s, Barker et al. (Reference Barker, Hales and Fall6) demonstrated that systolic blood pressure in older children is inversely related to their birth weight. In the 2000s, some epidemiological studies have confirmed that infants with low birth weight or intra-uterine growth restriction presented higher systolic and diastolic blood pressure than a control group as adults(Reference Fattal-Valevski, Bernheim and Leitner7–Reference Shankaran, Das and Bauer9). In a 2012 meta-analysis, low birth weight was associated with an increased OR (1·2) for the development of hypertension(Reference Mu, Wang and Sheng10). However, the mechanism by which low birth weight is linked to later hypertension is not yet well understood.
In experimental models, maternal low-protein diet during gestation and lactation is one of the most studied animal models of developmental origin of metabolic diseases(Reference Ozanne and Hales3, Reference Burdge, Lillycrop and Jackson11, Reference Fidalgo, Falcao-Tebas and Bento-Santos12). For example, adult rats submitted to a perinatal protein undernutrition (5 % protein) showed higher blood pressure, later glomerulogenesis and lower nephron numbers than their normoproteic controls(Reference Villar-Martini, Carvalho and Neves13). The later consequence of kidney injuries was associated with albuminuria and proteinuria, and these effects seem to be amplified with age(Reference Keijzer-Veen, Schrevel and Finken14). Maternal exposure to a low-protein diet (8 % of casein) during pregnancy and lactation induces growth restriction, age-dependent loss of glucose tolerance, insulin resistance and changes in lung structure and ventilatory function in adult offspring(Reference Ozanne and Hales3, Reference Karadag, Sakurai and Wang15, Reference Leandro, da Silva Ribeiro and Dos Santos16). Thus, it can be suggested that there is some association between maternal undernutrition-induced short-term effects in respiratory function and the age of appearance of hypertension in adulthood. Recently, studies(Reference Costa-Silva, Zoccal and Machado17–Reference Simms, Paton and Allen19) have proposed that changes in the generation or modulation of respiratory function can contribute to the development of arterial hypertension. Our previous studies(Reference Costa-Silva, Zoccal and Machado17, Reference Costa-Silva, Silva and Pedi20, Reference Costa-Silva, Zoccal and Machado21) have suggested that respiratory neurons located in the brainstem could modulate the cardiovascular system and that increased respiratory activity would lead to arterial hypertension via central pathways. Currently, it is believed that ventilatory responses elicited by the activation of these pathways are due to the intrinsic sensitivity to O2/CO2 and inputs from specific regions of the central nervous system and carotid bodies, which lead to increased respiratory drive(Reference Forster and Smith22–Reference Wong-Riley, Liu and Gao24). Aside from the respiratory-related chemoreceptor responses, acute stimulation of central or peripheral respiratory chemoreceptors by hypercapnia or hypoxia produces increases in sympathetic activity and arterial pressure (AP)(Reference Guyenet, Stornetta and Abbott25, Reference Moreira, Takakura and Colombari26).
In the present study, we investigate the short- and long-term effects of a maternal low-protein diet on basal pulmonary ventilation (VE) and ventilatory control elicited by respiratory chemoreflex activation. Because disorders in the mechanisms of control of breathing, such as in the respiratory chemoreflex, are potential risk factors for the development of hypertension(Reference Passino, Giannoni and Milli27, Reference Abdala, McBryde and Marina28), our hypothesis was that a higher respiratory frequency (RF) and chemosensitivity to O2/CO2 at 30 d of age could be a potential hidden basis for an increased arterial blood pressure at 90 d of age.
Methods
The experimental protocol was approved by the Ethical Committee of the Biological Sciences Centre (process no. 23 076·044454/2010-94), Federal University of Pernambuco, Brazil, and followed the Guidelines for the Care and Use of Laboratory Animals(Reference Bayne29).
Animals
A total of seventeen virgin female albino Wistar rats (Rattus norvegicus) were obtained from the Academic Centre of Vitoria de Santo Antao (CAV), Federal University of Pernambuco, Brazil. The animals were maintained at a room temperature of 22 ± 1°C with a controlled light–dark cycle (dark 18.00–06.00 hours). A standard laboratory chow diet (52 % carbohydrate, 21 % protein and 4 % lipids – Labina; Purina Agriband) and water were given ad libitum. At 90 d of age, rats were mated (two females for one male). The day on which spermatozoa were present in a vaginal smear was designated as the day of conception (day 1 of pregnancy). Pregnant rats were then transferred to individual cages. The following two groups were formed based on dietary manipulations: mothers fed a 17 % casein diet (normal-protein group, NP, n 8) and mothers fed an 8 % casein isoenergetic diet (low-protein group, LP, n 9) ad libitum. Offspring were standardised as litters of eight pups 48 h after birth. Male offspring were used in each litter and females were used only to standardise the size of each litter to eight pups. During the suckling period, the mothers continued to be provided with the experimental diet, either 8 % of casein or 17 % of casein. Diets were made at the Laboratory of Experimental Nutrition-CAV, Federal University of Pernambuco according to the American Institute of Nutrition –(AIN-93)(Reference Reeves, Nielsen and Fahey30). Casein had previously been analysed and showed 85 % purity (85 g protein for each 100 g casein). The diets were isoenergetic, and only the amount of protein was changed (Table 1). At weaning, three or four male offspring from each litter were randomly housed in collective cages and received a standard diet (Labina; Purina Agriband) ad libitum. At least two male offspring from each litter were used to evaluate either the short-term effects (at 30 d of age: NP, n 7 and LP, n 8) or the long-term effects (at 90 d of age: NP, n 7 and LP, n 8).
Table 1 Nutritional composition of the diets (g/100 g diet)
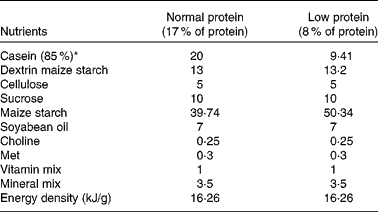
* Casein showed 85 % purity (85 g protein for each 100 g casein).
The body weight of pups was recorded at birth and on 30 and 90 d of age with an appropriate balance (model AS-1000; Marte), having an error range of 0·01 g. The body length of pups was recorded by the measurement of external surfaces (nose-to-anus length) with calipers (0·01 mm accuracy). The body weight and body length of pups were used to determine the anthropometrical parameter of the Lee index (cube root of body weight (g)/nose-to-anus length (cm)) and BMI (body weight (g)/square of nose-to-anus length (cm)).
Measurements of serum albumin, total protein, urea and creatinine
At 27 or 87 d of age, offspring from both groups were anaesthetised with ketamine (80 mg/kg) and xylazine (10 mg/kg), and blood samples (approximately 1 ml) were collected by plexus retro-orbital disruption. Serum samples were collected for the quantification of albumin, total protein, urea and creatininewith commercial kits (Labtest Diagnostica). Globulin values were obtained by subtracting albumin values from total protein values. The animals were fasted overnight.
Measurements of ventilation during basal, hypercapnia and hypoxia challenges
Measurements of VE were obtained at either 30 or 90 d of age using the whole-body plethysmography method as described by Malan(Reference Malan31). Before recording the data, the animals were placed into the Plexiglas chamber (5 litres) for a period of acclimatisation (approximately 60 min), and the chamber was flushed with humidified air and maintained at 25°C. Afterwards, to record the measurements of VE, air flow was suspended for short periods (3 min), and pressure oscillations caused by the breathing of the animals were captured by an apparatus connected to the chamber, with a pressure differential transducer and a signal amplifier (ML141 spirometer, PowerLab; ADInstruments). Then, the signal was captured into an acquisition system for data analysis (PowerLab; ADInstruments).
After recording the baseline VE measurements, the respiratory responses to CO2 were induced by flushing a hypercapnic gas mixture (7 % CO2, 21 % O2 and N2 balance; Linde Gas) into the plethysmographic chamber. This gas mixture was flushed into the chamber at a flow of 3 litres/min over the course of 5 min.
The respiratory responses to O2 were performed by flushing a hypoxic gas mixture (7 % O2 and N2 balance; Linde Gas) into the plethysmographic chamber. This gas mixture was flushed into the chamber at a flow of 3 litres/min over the course of 3 min, as previously standardised in our laboratory.
Analysis of respiratory parameters
RF, tidal volume (V T) and VE were determined in the room air condition (control baseline) and during the hypercapnic or hypoxic challenge. All data were analysed off-line with LabChart software (LabChart 7 Pro; ADInstruments). A period of 10 s was selected for the determination of mean RF. V T was calculated from the pressure oscillation caused by the breathing of rats and using the formula described previously by Malan(Reference Malan31). VE was obtained by multiplying RF by V T, and it is presented at ambient barometric pressure and at body temperature and pressure saturated.
Measurements of arterial blood pressure
At 48 h after the respiratory parameters were recorded, the NP and LP offspring (at 32 or 92 d of age) were anaesthetised with ketamine (80 mg/kg) and xylazine (10 mg/kg) for inserting a femoral arterial catheter (PE-50 connected to PE-10). The catheter was filled with heparinised saline (NaCl 0·9 %) and exteriorised through the animal's back. After surgery, the animals were injected with ketoprofen (5 mg/kg, intraperitoneally) and allowed to recover for 18 h before the beginning of the recording experiments. After this period, the arterial blood pressure and heart rate (HR) were recorded in unanaesthetised animals by connecting the femoral catheter to the pressure transducer. The signal was amplified (ML866/P, Power Lab; ADInstruments), sampled at 2 kHz, digitised and recorded in the microcomputer with appropriate software (LabChart 7 Pro; ADInstruments).
Each animal was placed in the recording chamber for a period of acclimatisation (approximately 60 min), pulsatile AP was recorded for 30 min at rest and the values of mean AP and HR were calculated from this recording period. For the determination of mean values of AP and HR, a period of 5–10 min was selected for each animal. All data were analysed off-line in appropriate software (LabChart 7 Pro; ADInstruments). At the end of the experiments, the animals were killed by decapitation.
Statistical analysis
Each experimental group included at least two animals from each litter. Bartlett's test was performed to evaluate data homogeneity in respiratory, cardiovascular, biochemical and murinometric parameters, and statistical results supported the use of a parametric test. Thus, significant differences between and within the groups were assessed by unpaired and paired Student's t tests, respectively. The significance level was fixed at P< 0·05. Data are expressed as means with their standard errors. Statistical analysis was performed using GraphPad Prism 5.0® software (GraphPad Software, Inc.).
Results
Pups from mothers submitted to a LP diet had lower birth weight, body length and BMI than the normoproteic group (Table 2). The reduced body weight in the LP group was maintained at 30 and 90 d of age, but with normalised values for BMI and the Lee index. In addition, there were no differences in the food intake between the groups at 30 d (NP 9·6 (sem 0·9) v. LP 9·7 (sem 0·9) g/100 g per 24 h) and 90 d (NP 9·3 (sem 0·6) v. LP 8·2 (sem 0·7) g/100 g per 24 h). Serum concentrations of albumin, total protein and globulin were reduced in the LP pups at 30 d of age but not at 90 d of age. Serum concentrations of urea and creatinine were increased at both 30 and 90 d of age.
Table 2 Somatic parameters (body weight, body length, BMI and Lee index) and serum biochemical parameters (albumin, globulin, total protein, urea and creatinine) of rats from dams submitted to a normoproteic (NP, 17 % of protein, n 14) or low-protein diet (LP, 8 % of protein, n 16) during pregnancy and lactation (Mean values with their standard errors)
* Mean values were significantly different from those of the NP group at each age (P< 0·05; unpaired Student's t test).
Breathing recordings of one representative rat from the NP and LP groups during the basal and hypercapnic conditions at 30 d and 90 d of age are shown in Fig. 1 ((a) and (b)). At 30 d of age, in the basal condition, the LP group had increased RF (Fig. 2(a)) and VE (Fig. 2(c)) when compared with the NP group, but there were no changes in V T at rest (Fig. 2(b)). At 90 d of age, in the basal condition, the LP group still showed elevated RF (Fig. 2(a)), but the V T and VE values had returned to normal levels (Fig. 2(b) and (c)). As expected, the activation of respiratory chemoreceptors by hypercapnia (CO2 7 %) produced an increase in RF, V T and VE values in both the NP and LP groups (Fig. 2). At 30 d of age, the LP group exhibited higher values of RF (Fig. 2(a1)) and VE (Fig. 2(c1)) during hypercapnia than did the NP group. No difference was found in V T values during hypercapnia (Fig. 2(b1)). At 90 d of age, the LP and NP groups had similar respiratory responses to hypercapnia (Fig. 2(a2), (b2) and (c2)).
Fig. 1 Representative tracings of rats at rest and during hypercapnia at 30 and 90 d of age. Representative tracing of ventilation before (before hypercapnia) and during the last minute of hypercapnia exposure (hypercapnia) in (a) 30- and (b) 90-d-old representative rats from dams submitted to either a normoproteic diet (NP group, 17 % of protein) or a low-protein diet (LP group, 8 % of protein) during pregnancy and lactation. All pups were fed a standard chow diet at weaning.
Fig. 2 Perinatal protein undernutrition increased ventilation (VE) at rest and during hypercapnia at 30 d and increased respiratory frequency (RF) at 90 d. Evaluation of (a) RF, (b) tidal volume (V T) and (c) VE before (basal) and after hypercapnia (7 % CO2) in 30- (a1, b1 and c1; normoproteic diet (NP), n 7 and HP, n 8) and 90-d-old rats (a2, b2 and c2; NP, n 7 and high-protein diet (HP), n 8) from dams submitted to a NP diet (17 % of protein, ■) or a LP diet (8 % of protein, ) during pregnancy and lactation. All pups were fed a standard chow diet at weaning. Values are means, with their standard errors represented by vertical bars. * Mean values were significantly different from those of the NP group in each condition (basal or hypercapnia) and at each age (30 or 90 d) (P< 0·05; unpaired Student's t test).
Breathing recordings of representative rats from the NP and LP groups during the basal and hypoxic conditions, at 30 and 90 d of age, are shown in Fig. 3((a) and (b)). At 30 d of age under the basal condition, the LP group had elevated RF (Fig. 4(a1)) and VE (Fig. 4(c1)) when compared with the NP group, but they showed no changes in V T values at rest (Fig. 4(b1)). At 90 d of age under the basal condition, the LP group still showed elevated RF (Fig. 4(a2)), but their V T and VE values had returned to normal levels (Fig. 4(b2) and (c2)). As expected, the activation of respiratory chemoreceptors by hypoxia (O2 7 %) produced an increase in RF, V T and VE values in both the NP and LP groups (Fig. 4). However, these responses were more pronounced in the LP pups at 30 d of age (Fig. 4(a1), (b1) and (c1)). At 90 d of age, both the groups responded at the same magnitude when stimulated by hypoxia (Fig. 4(a2), (b2) and (c2)).
Fig. 3 Representative tracings of rats at rest and during hypoxia at 30 and 90 d of age. Representative tracing of ventilation before (before hypoxia) and during the last minute of hypoxia exposure (hypoxia) in (a) 30- and (b) 90-d-old representative rats from dams submitted to a normoproteic diet (NP group, 17 % of protein) or a low-protein diet (LP group, 8 % of protein) during pregnancy and lactation. All pups were fed a standard chow diet at weaning.
Fig. 4 Perinatal protein undernutrition increased ventilation (VE) at rest and during hypoxia at 30 d and respiratory frequency (RF) at 90 d. Evaluation of (a) RF, (b) tidal volume (V T) and (c) VE, before (basal) and after hypoxia (7 % O2) in 30- (a1, b1 and c1; normoproteic diet (NP), n 7 and high-protein diet (HP), n 8) and 90-d-old rats (a2, b2 and c2; NP, n 7 and HP, n 8) from dams submitted to a NP (17 % of protein, ■) or a low-protein diet (LP, 8 % of protein, ) during pregnancy and lactation. All pups were fed a standard chow diet at weaning. Values are means, with their standard errors represented by vertical bars. * Mean values were significantly different from those of the NP group in each condition (basal or hypoxia) and at each age (30 or 90 d) (P< 0·05; unpaired Student's t test).
The pulsatile AP, mean AP and HR recordings of representative rats from the NP and LP groups at 30 and 90 d of age are shown in Fig. 5((a) and (b)). At 30 d of age, there were no differences between the groups in terms of baseline values of systolic AP, diastolic AP, mean AP and HR (Fig. 6(A)–(D), respectively). At 90 d of age, the levels of AP in the LP animals were significantly increased, but there were no changes in the levels of HR (Fig. 6).
Fig. 5 Representative tracings of baseline arterial pressure of rats at 30 and 90 d of age. Representative tracing of baseline pulsatile arterial pressure (PAP), mean arterial pressure (MAP) and heart rate (HR) in (a) 30- and (b) 90-d-old rats from dams submitted to a normoproteic (NP, 17 % of protein) or a low-protein diet (LP, 8 % of protein) during pregnancy and lactation. All pups were fed a standard chow diet at weaning.
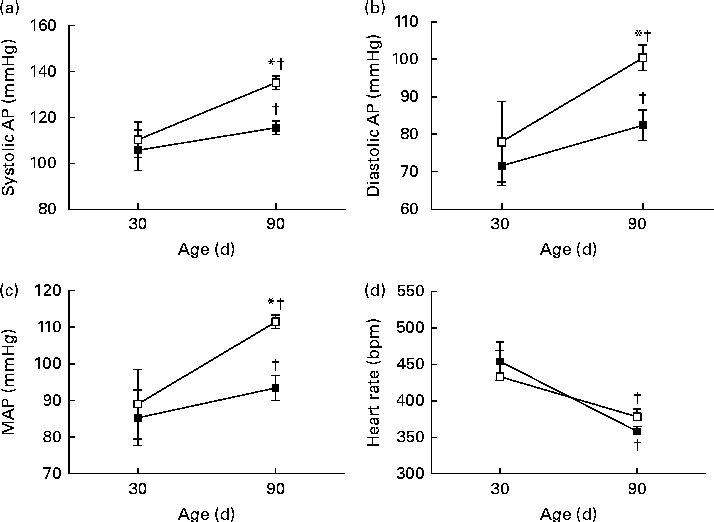
Fig. 6 Perinatal protein undernutrition increased arterial pressure at 90 d. Evaluation of (a) systolic arterial pressure (systolic AP), (b) diastolic arterial pressure (diastolic AP), (c) mean arterial pressure (MAP) and (d) heart rate in 30- and 90-d-old rats from dams submitted to a normoproteic (NP, 17 % of protein, n 13, ) or a low-protein diet (LP, 8 % of protein, n 9,
) during pregnancy and lactation. All pups were fed a standard chow diet at weaning. * Mean values were significantly different from those of the NP group at specific age (P< 0·05; unpaired Student's t test). † Mean values were significantly different from those at 30 d of age (P< 0·05; paired Student's t test).
Discussion
We investigated the short- and long-term effects of feeding a LP diet during gestation and lactation on basal pulmonary VE and ventilatory control elicited by respiratory chemoreflex activation. The main finding of the present study is that protein restriction during perinatal development differentially alters the VE and ventilatory responses to respiratory chemoreflex activation in an age-dependent manner. Actually, although there were differences between the control and perinatal LP animals in response to hypercapnia and hypoxia at 30 d of age, no changes were found in ventilatory responses at 90 d of age. However, since disorders in the mechanisms of control of breathing are potential risk factors for the development of hypertension(Reference Passino, Giannoni and Milli27, Reference Abdala, McBryde and Marina28), the high RF and chemosensitivity to O2/CO2 at 30 d of age were associated with increased arterial blood pressure at 90 d of age. These observations indicate that the short-term maternal LP diet induced changes in the ventilatory frequency. It can be suggested that there is a relationship between this early change and the onset of high blood pressure in adulthood.
Previous studies(Reference Lopes de Souza, Orozco-Solis and Grit32–Reference Orozco-Solis, Lopes de Souza and Barbosa Matos34) have demonstrated that perinatal LP diet exposure leads to lower weight in the offspring. Furthermore, maternal low protein intake during pregnancy leads to smaller birth values of growth indices such as body length, BMI and Lee index. A low supply of protein in the maternal diet during pregnancy could induce a deficit in the maternal–fetal amino acid transport through the placenta, leading to the restriction of fetal growth and producing a low birth weight(Reference Harding35, Reference Metcoff, Cole and Luff36). After birth, maternal protein restriction during lactation affects protein levels in the maternal milk(Reference Grigor, Allan and Carne37), which could reduce the protein intake of pups and produces a reduction of serum protein levels in the offspring at 30 d of age. It is interesting to note that serum urea and creatinine levels remained high in 90-d-old LP offspring, suggesting that renal excretion is permanently disturbed, as observed in previous studies(Reference Nwagwu, Cook and Langley-Evans38, Reference Harrison and Langley-Evans39).
Recent studies(Reference Costa-Silva, Zoccal and Machado17–Reference Simms, Paton and Allen19) have indicated that changes in the generation or modulation of respiratory function can contribute to the development of arterial hypertension. It has been shown that spontaneously hypertensive rats exhibited amplified respiratory–sympathetic coupling in early life and changes in baseline respiratory rhythm before developing hypertension(Reference Simms, Paton and Pickering18). Our previous studies(Reference Costa-Silva, Zoccal and Machado17, Reference Costa-Silva, Silva and Pedi20, Reference Costa-Silva, Zoccal and Machado21) have suggested that respiratory neurons located in the brainstem could modulate the cardiovascular system and that increased respiratory activity would lead to arterial hypertension via central pathways. Herein, we showed that maternal protein restriction during pregnancy and lactation leads to relevant short-term effects on the respiratory function of the offspring, such as increased baseline RF (up to 28 %) and VE (up to 40 %). These short-term effects elicited by maternal protein restriction are important findings and strongly suggest changes in respiratory–sympathetic coupling in early life(Reference Costa-Silva, Zoccal and Machado17, Reference Costa-Silva, Zoccal and Machado21), which would contribute to raising arterial blood pressure in the adult life of these animals.
Central or peripheral respiratory chemoreceptors play important roles in acid–base homeostasis and respiratory control. These cells are sensitive to changes in O2/CO2 levels in the body and enhance breathing in response to decreases in O2 levels or increases in CO2 levels(Reference Huckstepp and Dale23, Reference Guyenet, Stornetta and Bayliss40, Reference Wenker, Benoit and Chen41). In addition, acute stimulation of these respiratory chemoreceptors elicited by hypoxia or hypercapnia increases sympathetic activity and AP(Reference Forster and Smith22–Reference Guyenet, Stornetta and Abbott25, Reference Guyenet, Stornetta and Bayliss40). It is well established that the critical period for the development of the respiratory system includes the entirety of the fetal period as well as postnatal life until day 15 in rats(Reference Wong-Riley, Liu and Gao24, Reference Putnam, Conrad and Gdovin42, Reference Davis, Solhied and Castillo43). During this period, there is intense development and maturation of chemoreceptors and the respiratory network, and any environmental perturbations in that critical period can alter the development of the respiratory system(Reference Davis, Solhied and Castillo43). Herein, maternal protein restriction during pregnancy and lactation led to increased sensitisation of respiratory chemoreceptors in early age, with high ventilatory responses to hypoxia and hypercapnia. However, after exposure to a NP diet (after weaning), O2/CO2 chemosensitivity returned to normal levels in adulthood. These findings demonstrated that maternal protein undernutrition induces increased VE and O2/CO2 chemosensitivity in early life. In this context, a study by Abdala et al. (Reference Abdala, McBryde and Marina28) has proposed that hypertension is critically dependent on chemoreceptor input in hypertensive conditions and is modulated by the respiratory rhythm generator in juvenile rats(Reference Simms, Paton and Pickering18, Reference Zoccal, Simms and Bonagamba44, Reference Tan, Lu and Whiteis45). Thus, considering that offspring from protein-restricted dams had increased chemoreceptor sensitivity, the present data strongly suggest that increased arterial blood pressure in adulthood could be dependent on the short-term effects elicited by maternal protein restriction. This thereby provides insights into the mechanisms that underlie the development of arterial hypertension in perinatal protein-restricted individuals.
The effects on respiratory rhythm seem to be unchangeable and could be related to the changes in the gene expression of chemosensory transducers, modifying the cellular phenotype in chemosensitive cells(Reference Huckstepp and Dale23, Reference Tan, Lu and Whiteis45). These effects could induce permanent modifications in neuronal activity and the respiratory pattern generator as well as in O2/CO2 chemosensitivity in the brainstem. These abnormalities in gene expression of the organs and central nervous system of protein-restricted offspring are worthy of future investigation.
Studies performed in young offspring from protein-restricted dams have shown changes in gene expression involved in aspartate and glutamate metabolism(Reference McNeil, Hay and Rucklidge46), which play an important role in the excitatory neurotransmission of the central nervous system(Reference Rubio and Juiz47, Reference Fuchs, Berger and Klomp48). Recently, it has been demonstrated that rats submitted to malnutrition have altered central glutamatergic pathways and that these disruptions could be involved in the development of arterial hypertension in that experimental model(Reference Rodrigues, Chianca-Jr and Gonçalves Fernandes49). Furthermore, studies in hypertensive animals have shown the involvement of glutamatergic mechanisms in the brainstem(Reference Costa-Silva, Zoccal and Machado17, Reference Moraes, Zoccal and Machado50, Reference Moraes, Zoccal and Machado51) and a decreased expression of genes encoding antioxidant enzymes and an increased expression of pro-oxidant enzymes, leading to elevated oxidative stress and increased AP(Reference Nanduri, Makarenko and Reddy52). This suggests that genes related to redox homeostasis could also be important keys to understanding the mechanisms underlying the development of hypertension. In addition, studies have suggested that maternal protein undernutrition could induce increases in the expression of hypoxia-inducible factor 1 α in the offspring and could be a risk factor for the development of arterial hypertension(Reference Ito, Funamoto and Sato53–Reference Lee, Wolf and Escudero56).
In conclusion, the present data showed that short-term effects induced by a protein-restricted diet during the perinatal period included increased respiratory rhythm and O2/CO2 chemosensitivity, which could contribute to the development of long-term effects, such as increased AP in adulthood. These findings provide insights into the effects of maternal protein restriction and suggest that increased O2/CO2 chemosensitivity is one possible risk factor for the development of arterial hypertension in perinatal protein-restricted animals.
Acknowledgements
The authors acknowledge Dr Carmen de Castro Chaves for experimental support and discussion during the development of this work.
The present study was supported by the Fundação de Amparo a Ciência e Tecnologia de Pernambuco (FACEPE, grant no. 1365-2.07/10) and the Conselho Nacional de Desenvolvimento Científico e Tecnológico (CNPq, grant no. 484452/2011-8). FACEPE and CNPq had no role in the design, analysis or writing of this article.
J. L. d. B. A., V. O. N., C. G. L. and J. H. C.-S. contributed to the conception and design of the study, the collection, analysis and interpretation of the data, and the drafting or revising of the manuscript; G. B. d. O. contributed to the collection, analysis and interpretation of the data; G. S. F. d. S. and A. G. W. contributed to the collection, analysis and interpretation of the data and the drafting or revising of the manuscript.
None of the authors has any conflicts of interest.