The development of sustainable aquaculture is partly dependent on sustainable aquafeeds and the replacement of some or all of the protein and oil derived from marine sources( Reference Miller, Nichols and Carter 1 – Reference Bostock, McAndrew and Richards 3 ). Strategies include the use of protein and oil sources derived from agriculture production as well as consideration of organic and certified ingredients that may include those of marine origin( Reference Hardy 4 , Reference Mente, Karalazos and Karapanagiotidis 5 ). While considerable knowledge exists about the use of alternative protein sources in aquafeeds, the majority of this has been obtained from long-term growth experiments. Protein turnover is clearly of central importance to growth but only a few studies on fish have investigated protein turnover( Reference Vilhelmsson, Martin and Medale 6 ), protein synthesis and protein degradation, in relation to dietary modification; even fewer have investigated the effect of replacing fishmeal on protein synthesis( Reference De la Higuera, Akharbach and Hidalgo 7 – Reference Langer, Guillaume and Metailler 10 ). Protein turnover reflects dietary protein in relation to how closely it matches quantitative and qualitative amino acid requirements( Reference Carter and Houlihan 11 – Reference Panserat and Kaushik 13 ). Low or imbalanced amino acid supply tends to initially stimulate protein synthesis in the liver in order to maintain protein synthesis and growth in the skeletal muscle( Reference Langer and Guillaume 9 ). Prolonged feeding at a low intake of amino acids will down-regulate protein synthesis so that liver rates decrease to be the same or lower than on a higher-quality protein source and skeletal muscle protein synthesis cannot be sustained and will be depressed( Reference Carter and Houlihan 11 , Reference Peragon, Barroso and Garcia-Salguero 14 ). When an alternative dietary protein is of high quality, muscle protein synthesis may not be affected by protein sources used, as in barramundi (Lates calcarifer) fed lupin meal to replace 45 % of the fishmeal protein( Reference Katersky and Carter 15 ). In contrast, whole-animal protein synthesis differed between rainbow trout (Oncorhynchus mykiss) fed two isonitrogenous diets but with varying amounts of fishmeal and other protein sources( Reference Martin, Vilhelmsson and Medale 16 ).
Postprandial changes in protein synthesis determine a large proportion of specific dynamic action( Reference Lyndon, Houlihan and Hall 17 , Reference McCarthy and Fuiman 18 ) and are influenced by nutritional factors including feed intake and diet composition. Peak rates of protein synthesis generally occur between 4 and 12 h after a meal and measurement of protein synthesis has been proposed as a potentially sensitive method to compare diets and explain some nutritional effects( Reference Carter and Houlihan 11 , Reference Millward 19 ). Very few studies have compared the combined effects of diet and time after feeding on fish protein synthesis in vivo. In the aforementioned study on barramundi fed the lupin meal( Reference Katersky and Carter 15 ), protein synthesis was not affected by protein source at peak (4 h) or at basal (24 h) rates. It was therefore of interest to further investigate the effect of alternate protein sources on protein synthesis in the present study on gilthead sea bream (Sparus aurata L.) and at greater resolution than in any previous research on fish by considering multiple times following feeding. This is relevant in that plant proteins are likely to alter tissue free amino acid pools( Reference Gomez-Requeni, Mingarro and Calduch-Giner 20 ) and the time of peak postprandial digestive enzyme activity( Reference Santigosa, Garcia-Meilan and Valentin 21 ) in sea bream. Several potential alternate proteins have been successfully tested with sea bream and inclusion of 30 % of soyabean did not significantly affect growth, feed or protein efficiency( Reference Robaina, Izquirdo and Moyano 22 – Reference Nengas, Alexis and Davis 24 ) and was selected as the primary not significantly affect growth, feed or protein efficiency alternative to fishmeal in the present study.
The principal aim of the present study was to assess postprandial protein synthesis as a sensitive test of nutritionally similar diets with different ingredient composition. Measurement of protein synthesis using a flooding dose of a labelled amino acid( Reference Garlick, McNurlan and Preedy 25 ) has advanced the understanding of the relationships between diet composition, protein metabolism and nutrient utilisation in aquatic animals and is the method that has been used most extensively in fish( Reference De la Higuera, Garzon and Hidalgo 8 , Reference Langer and Guillaume 9 , Reference Carter, Houlihan and Brechin 26 ). However, the complexity of the method means that there are relatively few published studies on fish that compare diets( Reference Carter and Houlihan 11 , Reference Fraser and Rogers 27 ). Consequently, new research adds considerably to the existing pool of information. Protein metabolism was measured before feeding and at five times after feeding over a period of 48 h in order to make a detailed comparison of partial fishmeal replacement with soyabean. A third diet was then used to assess whether measuring protein synthesis at three critical times provided sufficient resolution of postprandial changes in order to validate the optimum measurements.
Methods
Experimental diets
In the present study, three isonitrogenous diets were formulated to compare the effect of partial fishmeal replacement (Table 1): one used a sustainable certified Peruvian fishmeal (FM63); the second used soyabean protein to replace approximately 16% of the fishmeal protein (FM55); and the third used soyabean and maize gluten protein to replace approximately 27% of the fishmeal protein (FM50). Ingredients were sourced from the Zoonomi Aquafeed Company. Diets were prepared at the Fish Nutrition and Pathology Laboratory, Institute of Aquaculture, Hellenic Centre for Marine Research.
Table 1 Ingredient and chemical composition of the three partial fishmeal replacement experimental diets (FM63, FM55 and FM50)
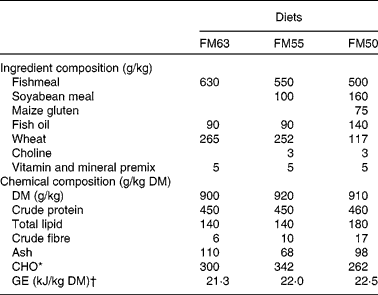
CHO, carbohydrate; GE, gross energy.
* CHO calculated by difference (100 % − (crude protein (%)+total lipid (%)+ash (%)).
† GE calculated from nutrients assuming a GE content of 23·6 kJ/g for protein, 39·5 kJ/g for lipid and 17·2 kJ/g for carbohydrate( Reference Brafield 46 ).
Growth experiment
Gilthead sea bream were obtained from a commercial fish farm (SE Saronic Gulf) and reared at the Fish Nutrition and Pathology Laboratory, Institute of Aquaculture (Hellenic Centre for Marine Research). Care and use of animals was according to the institutional procedures at the Hellenic Centre for Marine Research. Fish were held in 2 m3 experimental net cages (1 m × 2 m × 1 m deep) suspended in a concrete raceway supplied continuously with filtered seawater; there were three cages per treatment and these were each stocked with twenty fish. Fish were fed ad libitum by hand three times daily at 09.00, 12.00 and 15.00 hours for 35 d to measure feed intake and growth. At the end of this period, fish were used to measure liver and white muscle protein synthesis before feeding and at up to five times after feeding (see below). Fish were killed by anaesthesia (diluted 1:1 phenoxyethanol in ethanol) and a blow to the head, wet weight (W) was measured and the tissues rapidly dissected out, samples weighed and frozen in liquid N2. W was measured in all the remaining fish not used for protein synthesis. The hepatic somatic index (HSI; % weight) was calculated as HSI = 100 × (liver weight/W) where liver weight and W are in g.
Protein synthesis
Liver and white muscle rates of protein synthesis were measured following a single flooding dose of [3H]phenylalanine( Reference Garlick, McNurlan and Preedy 25 , Reference Houlihan, Carter and McCarthy 28 ) before feeding, and at 4, 8, 12, 24 and 48 h after feeding; protein synthesis was not measured at 8 and 12 h for fish fed FM55. Replicate samples, taken at 28, 55, 71, 110 and 176 min, were used to establish a time course and validate the method. Fish were injected into the caudal vein with 1·0 ml/100 g injection solution. Following injection, fish were returned to tanks in an indoor system held under constant environmental conditions and containing aerated seawater; they were removed and killed after a known time. The injection solution contained 150 mmol l-phenylalanine and l-[2,6-3H]phenylalanine (Amersham Pharmacia Biotech) in 0·2 μm filtered seawater at pH 7·4 with a measured specific activity of 1473 (se 252) dpm/nmol phenylalanine. The treatment of samples to measure protein-bound and free-pool phenylalanine-specific radioactivity was as described previously for fish( Reference Houlihan, Carter and McCarthy 28 ). Fractional rates of protein synthesis (k s: %/d) were calculated as k s = 100 × ((S b/S a) × (1440/t 1)), where S b is the protein-bound phenylalanine-specific radioactivity at time t 1 (min) and S a the free-pool phenylalanine-specific radioactivity( Reference Garlick, McNurlan and Preedy 25 , Reference McNurlan, Tomkins and Garlick 29 , Reference Garlick, Fern and Preedy 30 ). Protein concentration was measured using a modification of the Folin phenol method( Reference Lowry, Rosebrough and Farr 31 ) and RNA concentration was measured using dual-wavelength absorbance( Reference Ashford and Pain 32 ). RNA was also expressed as the capacity for protein synthesis (C s: mg RNA/g protein) and as RNA activity (k RNA: k s/g RNA per d)( Reference Sugden and Fuller 33 ).
Statistical analysis
Data are presented as means with their standard errors. Homogeneity was confirmed using Levene's test. One-way ANOVA and linear regression were used to analyse the relationship between incorporation time and both free-pool and protein-bound phenylalanine-specific radioactivity for each tissue( Reference Garlick, McNurlan and Preedy 25 ). Two-way ANOVA was used to analyse the effect of diet and time after feeding on protein synthesis and capacity for protein synthesis. When there was no significant interaction between the two factors, the main effects were analysed. Significance was accepted at 5 % or less. All statistical analyses were carried out using IBM SPSS Statistics, version 19.
Results
Growth performance
Diet had no effect on the growth performance of sea bream: there were no significant differences among the diets for final weight, weight gain, specific growth rate, feed intake and feed efficiency ratio (Table 2). Liver weight was significantly higher in fish fed FM55 (0·840 (se 0·031) g) compared with those fed FM63 (0·705 (se 0·037) g) or FM50 (0·724 (se 0·037) g). Accounting for W, the HSI was significantly lower for fish fed FM50 (1·07 (se 0·05) % W) compared with those fed FM55 (1·27 (se 0·04) % W); fish fed FM63 (1·25 (se 0·06) % W) were not different from either of the other two diets. However, liver protein whether expressed as concentration or total protein was not different among the diets.
Table 2 Morphometric data for sea bream fed diets with differing fishmeal content (Mean values with their standard errors, n 3)

FM63, 63 % fishmeal; FM55, 55 % fishmeal with approximately 16 % fishmeal protein replaced by soyabean protein; FM50, 50 % fishmeal with approximately 27 % fishmeal protein replaced by soyabean and maize gluten protein; SGR, specific growth rate; FER, feed efficiency ratio.
* SGR = 100 × ((Ln (final weight/initial weight))/35 d).
† FER = feed intake (g)/weight gain (g).
Validation of flooding dose
The time course for the incorporation of [3H]phenylalanine showed that the flooding-dose technique is suitable for the study of protein turnover in sea bream (Fig. 1). Incorporation into the liver and white muscle was measured between 28 and 176 min following injection and increased over the range of incorporation times (ANOVA, P < 0·05). The free phenylalanine concentration in the white muscle free pool was 388 (se 41) nmol phenylalanine/g wet mass. Using a white muscle free phenylalanine concentration of 150 nmol phenylalanine/g wet mass in uninjected fish, there was more than a 2-fold increase in free phenylalanine following injection.

Fig. 1 Time course for phenylalanine-specific radioactivity (dpm/nmol phenylalanine (phe)) in (a) liver free pool (significant differences between 28 and 176 min: F 4,7 = 5·36, P = 0·027); (b) liver protein, as described by S b = 0·115 × (0·012)t+0·361 × (1·271) (R 2 0·90, F 1,10 = 99·7, P < 0·001); (c) white muscle free pool (no differences between times: F 4,7 = 0·86, P = 0·53); (d) white muscle protein, as described by S b = 0·009 × (0·002)t − 0·201 × (0·202) (R 2 0·67, F 1,10 = 23·34, P < 0·001). Values are means, with their standard errors represented by vertical bars.
There was a significant difference between liver free-pool (S a) phenylalanine-specific radioactivity (dpm/nmol phenylalanine) at different times (F 4,7 = 5·363, P = 0·027) (Fig. 1(a)). This was due to S a being significantly higher at 110 min than at 176 min. The decrease in S a at 176 min caused a weak linear relationship between incorporation time and liver free-pool (S a) phenylalanine-specific radioactivity described by S a = 1235 − 3·196t (R 2 0·276, F 1,10 = 5·187, P = 0·046); there was no relationship when the 176 min data were excluded. Over the incorporation time, there was a positive linear relationship between time and protein-bound (S b) phenylalanine-specific radioactivity (dpm/nmol phenylalanine) described by S b = 0·115t+0·361 (R 2 0·900, F 1,10 = 99·7, P < 0·001) (Fig. 1(b)). Thus, the flooding-dose method was valid for incorporation times of between 28 and 110 min in the liver.
There were no significant differences between white muscle free-pool (S a) phenylalanine-specific radioactivity (dpm/nmol phenylalanine) at different times (F 4,7 = 0·863, P = 0·530) and white muscle free pools therefore remained flooded for at least 176 min. The free-pool S a in the white muscle was 936 (se 91) dpm/nmol phenylalanine, 64 % of the injection solution (Fig. 1(c)). Over the time course, there was a linear relationship between time and protein-bound (S b) phenylalanine-specific radioactivity (dpm/nmol phenylalanine) described by S b = 0·009t − 0·201 (R 2 0·67, F 1,10 = 23·3, P < 0·001) (Fig. 1(d)). Thus, the flooding-dose method was valid for incorporation times of between 28 and 176 min in the white muscle.
Postprandial protein metabolism
Detailed examination of postprandial protein metabolism was made between diets FM63 and FM50 by measuring protein synthesis and capacity for protein synthesis before and at 4, 8, 12, 24 and 48 h after feeding one meal. Liver protein synthesis was not different (F 1,36 = 0·146, P = 0·704) between the two diets, whereas there were significant differences (F 5,36 = 8·245, P < 0·001) due to time. Liver rates of protein synthesis were significantly lower before feeding and at 24 and 48 h after feeding than at 4 and 8 h after feeding (Fig. 2(a)). White muscle protein synthesis had similar responses; it was not affected by diet (F 1,36 = 0·314, P = 0·579) but only by time (F 5,36 = 7·000, P < 0·001); rates were significantly lower before and at 24 and 48 h after feeding than at 4 and 8 h after feeding (Fig. 2(b)). Thus, the peak rates of protein synthesis were similar in the two tissues and across the two diets. In contrast, the capacity for protein synthesis was not affected by diet or by time in either tissue; the overall mean values were 81·02 (se 1·682) and 4·147 (se 0·147) mg RNA/g protein for the liver and white muscle, respectively.

Fig. 2 Protein synthesis (%/d) in (a) liver and (b) white muscle of sea bream before feeding and at different times after feeding. Values are means, with their standard errors represented by vertical bars (n 8).
Diet comparison using protein metabolism
Protein metabolism of fish fed FM55 was compared with diets FM63 and FM50 at selected times (0, 4, 24 and 48 h) to examine whether there were any differences due to diet or time after feeding. Diet did not influence protein synthesis or the capacity for protein synthesis in either tissue. However, time after feeding had a significant effect on protein synthesis. At 4 h, protein synthesis was higher than at the other three times for all diets and for both liver (Table 3) and white muscle (Table 4). Absolute liver protein synthesis was significantly lower in fish fed FM55 (0·022 (se 0·005) g protein) than in those fed FM50 (0·027 (se 0·003) g protein) or FM63 (0·026 (se 0·002) g protein). Neither time nor diet influenced the capacity for protein synthesis; the overall mean values were 81·021 (se 1·682) mg RNA/g protein and 4·066 (se 0·943) mg RNA/g protein for the liver and white muscle, respectively.
Table 3 Liver fractional rates of protein synthesis (k s: %/d) for sea bream fed diets with differing fishmeal content before feeding (0 h) and at different times after feeding (Mean values with their standard errors, n 4)

FM63, 63 % fishmeal; FM55, 55 % fishmeal with approximately 16 % fishmeal protein replaced by soyabean protein; FM50, 50 % fishmeal with approximately 27 % fishmeal protein replaced by soyabean and maize gluten protein.
a,b Mean values with the same superscript letters across time when combined by diet were not significantly different following two-way ANOVA and multiple comparison by Tukey's honestly significant difference.
Table 4 White muscle fractional rates of protein synthesis (k s: %/d) for sea bream fed diets with differing fishmeal content before feeding and at different times after feeding (Mean values with their standard errors, n 4)

FM63, 63 % fishmeal; FM55, 55 % fishmeal with approximately 16 % fishmeal protein replaced by soyabean protein; FM50, 50 % fishmeal with approximately 27 % fishmeal protein replaced by soyabean and maize gluten protein.
a,b Mean values with the same superscript letters across time when combined by diet were not significantly different following two-way ANOVA and multiple comparison by Tukey's honestly significant difference.
There was individual variation in the indices of protein metabolism and a positive correlation between the liver and white muscle fractional rates of protein synthesis (r 0·477; P < 0·01; n 60) and the tissues' RNA activity (r 0·497; P < 0·01; n 60). There was no correlation between the tissues' capacity for protein synthesis. Thus, individual variation in protein metabolism was via changes in RNA activity rather than RNA content.
Discussion
The main result from the present study was the similarity in measures of protein metabolism among fish fed three isonitrogenous diets with different levels of fishmeal and other protein sources. The measurement of protein metabolism was comprehensive and focused on the two key tissues, the liver and white muscle, and at different times after feeding. Postprandial protein synthesis peaked at 4 h in both tissues and remained elevated for at least 4 h before returning to pre-feeding levels after 24 h. The measures of protein metabolism were mainly made within a 24 h daily cycle and the absence of a diet effect was reflected by the similarity in long-term measures of growth performance. Interestingly, diet had a subtle influence on the liver so that the overall effect was fish fed FM55 synthesised less liver protein despite similar fractional rates of protein synthesis. Capacity for protein synthesis did not change following feeding and showed that the changes in protein synthesis were primarily due to the changes in RNA activity in both tissues. Thus, the pattern of postprandial protein synthesis in sea bream was broadly similar to other warm-water fish species( Reference McCarthy and Fuiman 18 , Reference Katersky and Carter 34 ) and to when nutritionally similar diets were fed to previously well-fed fish( Reference Katersky and Carter 15 ).
Postprandial protein synthesis
Postprandial metabolism, often termed specific dynamic action or heat increment, reflects the many physiological changes stimulated by a meal( Reference McCue 35 ). As with other animals, protein synthesis accounts for a large part of postprandial energy expenditure in fish and emphasises the importance of understanding postprandial protein synthesis( Reference Lyndon, Houlihan and Hall 17 , Reference McCarthy and Fuiman 18 , Reference Brown and Cameron 36 ). To our knowledge, the in vivo effect of diet on postprandial protein synthesis in fish has been measured by only Katersky & Carter( Reference Katersky and Carter 15 ). Sea bream in the present study increased white muscle rates by over five times during the peak at 4 and 8 h after feeding; protein synthesis peaked in the liver at the same time and increased by about 1·7 times. In warm-water fish, there appears to be closer alignment between peak activity in the liver and white muscle( Reference Katersky and Carter 34 ), whereas the liver peak rate precedes the white muscle peak in colder-water species( Reference Carter and Houlihan 11 ). The relative increase is typically larger in the liver than in the white muscle( Reference Carter and Houlihan 11 ). This has been explained by the liver's primary role in primary processing of ingested nutrients and exportation of amino acids and synthesised proteins from the liver to peripheral tissues( Reference Peragon, Barroso and Garcia-Salguero 14 , Reference McCarthy and Fuiman 18 , Reference Carter, He and Houlihan 37 ). In contrast, stimulation of peak protein synthesis in sea bream white muscle was higher than for the liver, whereas the relative increase was similar between the two tissues for barramundi( Reference Katersky and Carter 34 ). The explanation may be due to a complex mix of differences between experiments that include fish species, age and size as well as to differences in dietary and environmental factors.
Dietary protein and protein synthesis
In the present study, sea bream were fed three nutritionally balanced diets that provided optimum protein within the range of 40–48 %( Reference Company, Calduch-Giner and Kaushik 38 – Reference Vergara, Robaina and Izquierdo 40 ) at an optimum protein:energy ratio of about 28·5 g dietary crude protein/dietary energy( Reference Lupatsch, Kissil and Sklan 41 ). They were formulated to replace some fishmeal with alternative protein sources, and sea bream showed very similar growth performance that was matched by indices of protein metabolism. The similarity in responses was therefore not unexpected but the present study is the first published study on fish to directly compare dietary effects on postprandial protein synthesis at multiple time points following feeding. In the few studies on fish that compared diets, protein synthesis was usually measured at a single time point after feeding( Reference Langer, Guillaume and Metailler 10 , Reference Carter, Houlihan and Brechin 26 ) that was often not specified( Reference De la Higuera, Garzon and Hidalgo 8 , Reference Peragon, Barroso and Garcia-Salguero 42 , Reference Sveier, Raae and Lied 43 ). To account for specific dynamic action, protein synthesis was measured at peak (4 h) and baseline (24 h) rates in barramundi( Reference Katersky and Carter 15 ).
Differences in dietary amino acid balance of alternative protein sources partly explain decreased long-term growth performance, and these differences are also highlighted by differences in protein turnover( Reference Langer and Guillaume 9 , Reference Langer, Guillaume and Metailler 10 ). At a physiological level and as a method, protein synthesis effectively integrates amino acid supply to provide an assessment of protein quality( Reference Carter and Houlihan 11 , Reference Young, Yu and Krempf 44 ). Diets that provide a poor amino acid balance tend to stimulate liver protein synthesis( Reference De la Higuera, Garzon and Hidalgo 8 ) and depress white muscle protein synthesis( Reference De la Higuera, Akharbach and Hidalgo 7 ). At the whole animal level, diets with poorer amino acid balance stimulated protein synthesis( Reference Langer and Guillaume 9 , Reference Langer, Guillaume and Metailler 10 ); this effect was because protein synthesis was measured at 3 h after feeding when, presumably, peak rates of liver protein synthesis accounted for a large proportion of total protein synthesised. At peak rates, liver protein synthesis can often account for most of whole-fish protein synthesis( Reference Katersky and Carter 34 ). In addition to time after feeding, response also depends on the extent of amino acid deficiency in a way that reflects the interaction between dietary protein content and quality in relation to requirements( Reference Carter and Houlihan 11 ). At marginal amino acid deficiency, on a marginally deficient diet or after a short length of time on a deficient diet, fish compensate and protein metabolism is stimulated in the liver in order to maintain muscle protein synthesis and growth( Reference Peragon, Barroso and Garcia-Salguero 14 ). Where the deficiency is more extreme, due to a more deficient diet or after a longer time, protein metabolism will down-regulate so that liver rates are similar or depressed and white muscle rates are depressed( Reference De la Higuera, Akharbach and Hidalgo 7 , Reference Peragon, Barroso and Garcia-Salguero 42 ). This suggests that measuring protein synthesis in the liver after a short period of time will highlight up-regulation due to amino acid deficiency, whereas measuring white muscle and whole-animal protein synthesis at the end of a growth experiment will explain the cause of differences in growth due to differences in protein turnover.
The present study suggested that there were some dietary differences in the liver. The liver size of fish fed FM55 was larger but liver protein concentration was lower, which meant that the total liver protein was not statistically different among the three diets. In contrast, total liver protein synthesis was significantly lower for FM55 despite there being no difference among the fractional rates of liver protein synthesis. The fish liver is sensitive to dietary differences and has a high capacity to compensate for some nutritional imbalances in order to optimise white muscle protein turnover and prioritise protein growth( Reference Peragon, Barroso and Garcia-Salguero 14 , Reference Viaplana-Marin, Fernandez-Borras and Blasco 45 ). In the present study, fish fed FM55 synthesised 84 % of the total protein compared with the other diets. Similarly, brown trout (Salmo trutta) fed a control diet synthesised 88 % of the total liver protein compared with those fed a slightly less adequate diet with higher carbohydrate and the need for greater export of liver proteins for muscle growth( Reference Viaplana-Marin, Fernandez-Borras and Blasco 45 ). Further research would be required to demonstrate whether FM55 was the better diet of the three tested.
An aim of much fish nutrition research is to replace fishmeal protein with alternative protein sources and, as in the present study, this generally requires the use of more than one alternative source. The measurement of protein synthesis can inform this process by highlighting differences between diets; as discussed above, the differences may be subtle and the measurement of protein synthesis in different tissues and at different times following feeding has the potential to highlight these. Lupin provides an excellent fishmeal replacement and 45 % replacement of fishmeal with lupin meal had no effect on white muscle protein synthesis at 4 or 24 h following feeding( Reference Katersky and Carter 15 ). Rainbow trout fed two different diets, both with mixed protein sources, had similar growth after 12 weeks, but there were differences in protein synthesis( Reference Martin, Vilhelmsson and Medale 16 ) which might suggest that a significant growth difference would become apparent after a longer time or at the same ration. The diets used for juvenile sea bream in the present study were formulated to reflect optimum protein supply and the similarity in growth and protein metabolism supports the use of alternative protein sources to replace part of the fishmeal.
Acknowledgements
The authors wish to express their thanks to G. Varvatsoulis and the Zoonomi Aquafeed Company for their help with feeds and to Panagiotis Ex and Antigoni Vasilaki for assistance with fish husbandry. Funding from the European Commission and the Hellenic Ministry of Rural Development and Food is gratefully acknowledged. The assistance of a member of the Editorial Board is gratefully acknowledged. All authors contributed to all parts of the research. The authors declare that there are no conflicts of interest.