Microbial populations residing in the human gut play a pivotal role in the host’s overall health by providing defence against pathogens, aiding in nutrient processing, lowering serum cholesterol level and improving the host’s immune functions (Fig. 1)(Reference Flint, Scott and Louis1–Reference Gotteland, Andrews and Toledo3). There are several factors that can lead to inter individual variations in gut microbial composition such as genetic factors, age, diet, the use of antibiotics and consumption of pre- and probiotics which can affect the colonisation of host gut microbiota(Reference Aravind, Wichienchot and Tsao4,Reference Kumar Singh, Cabral and Kumar5) . Such inter individual differences have been shown to affect the metabolism of ingested polyphenols(Reference Liu, Vervoort and Beekmann6). Dysbiosis in normal gut microbiota population can lead to chronic inflammatory conditions, for example, gastritis, inflammatory bowel syndrome, diarrhoea and colorectal cancer. Further, poor dietary habits, lack of exercise, stress and drugs can induce and sustain dramatic changes in the gut community structure(Reference Kumar Singh, Cabral and Kumar5). This can trigger a wide range of non-communicable diseases, including neurodegenerative diseases,CVD and obesity, some of which are combined in the metabolic syndrome(Reference Masumoto, Terao and Yamamoto7,Reference Sampson, Debelius and Thron8) . The association between specific microbial taxa and specific beneficial or harmful health outcomes continues to be an area of active research(Reference Duda-Chodak, Tarko and Satora9).

Fig. 1. Role of gut microbiota in affecting host health.
Flavonoids represent a major group of secondary metabolites found in several dietary sources including fruits, vegetables and drinks like coffee, tea and wine(Reference Vinson, Su and Zubik10). Flavonoids are well recognised for their potential anti-carcinogenic, antioxidant, anti-microbial and anti-inflammation effects(Reference Kumar and Pandey11,Reference Farkas, Jakus and Héberger12) . Additionally, flavonoids can mitigate against several degenerative diseases such as diabetes, obesity, CVD and neurodegenerative disease, or combinations such as metabolic syndrome(Reference Khurana, Venkataraman and Hollingsworth13). Possible beneficial effects of flavonoids depend mainly on their intrinsic bioavailability and vary between different flavonoids subclasses(Reference Manach, Williamson and Morand14). Generally, phenolic glycosides are not hydrolysed in the stomach(Reference Braune and Blaut15). Although some special aglycone moieties, depending on the structure, can show (other) reactions under the high HCl levels of the stomach, this is a rare case and not the focus of this review. Thus, after stomach passage, flavonoid glycosides are either hydrolysed in the small intestine by specific enzymes (e.g. lactase phlorizin hydrolase or human β-glucosidase(Reference Day, Cañada and Díaz16,Reference Day, DuPont and Ridley17) ) or metabolised in the large intestine by the action of intestinal microbiota to yield aglycones, the hydrophobic core of the flavonoid molecule(Reference Manach, Williamson and Morand14,Reference Manach, Scalbert and Morand18,Reference Blaut, Schoefer and Braune19) . Aglycones pass through cell membranes to exert their biological functions via passive diffusion(Reference Aherne and O’Brien20).
In addition, flavonoid ingestion can also modulate the gut microbial community(Reference Selma, Espin and Tomas-Barberan21). As such, dietary flavonoids and commensal gut microbiota exhibit a two-way mutual interaction. Firstly, flavonoids are metabolised by intestinal microbiota that leads to enhancement of their bioavailability, and secondly flavonoids can modulate the intestinal microbial population structure(Reference Lee, Jenner and Low22,Reference Murota, Nakamura and Uehara23) .
This review aims to provide a holistic overview on the interaction between dietary flavonoids in their different forms or classes and human intestinal microbiota. We start by describing the structure and distribution of the various classes of flavonoids in human diet. We then proceed to examine each major class of flavonoids in detail. Specifically, we examine their metabolic fate, structure activity relationship and role of the gut microbial communities in modulating their structure and activity, their impact on the microbial community structure inside the gut, and their overall impact on the host’s physiology.
Flavonoid classes
Flavonoids structure is based on a 15-carbon skeleton consisting of two phenyl rings (A and B) linked via a 3-carbon bridge forming a heterocyclic pyrane ring (C)(Reference Kumar and Pandey11,Reference Tsao24) . According to the substitution pattern on the central pyran ring, flavonoids are sub-classified into flavonols, flavones, flavanones, flavan-3-ols, isoflavones and anthocyanins, cf. (Fig. 2)(Reference Tsao24–Reference Lu, Xiao and Zhang26). Common dietary flavonoids include flavonols in onions, apples, and tea, flavanones in citrus fruits, flavan-3-ols in cocoa, tea, apples, and broad beans, and anthocyanins in berries and roses(Reference Williamson27). Only 5–10 % of the ingested flavonoids are absorbed in the small intestine, with the rest undergoing enzymatic breakdown by the resident microbiota in the large intestine or excretion(Reference Faria, Fernandes and Norberto28). Microbial β-glucosidases produced by specific gut microbes such as Bifidobaterium spp. and Lactobacillus spp. effectively hydrolyse flavonoid glycosides yielding aglycones and glucose units. Indeed, the amount of sugars released from phenolics is considered much lower compared with that from macronutrients such as pectin, etc. Glucose supports bacterial growth and is fermented to SCFA, the production of which triggers a wide range of beneficial physiological effects for the host, see Fig. 3(Reference Aravind, Wichienchot and Tsao4,Reference Faria, Fernandes and Norberto28) .
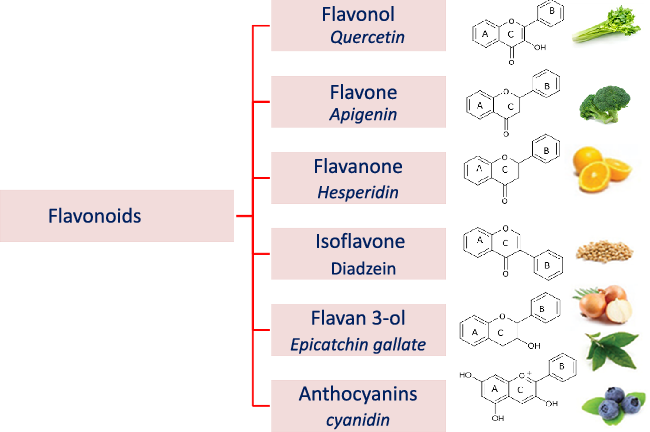
Fig. 2. Flavonoid subclasses (as structures) and example names of a common flavonoid of the respective subgroup.
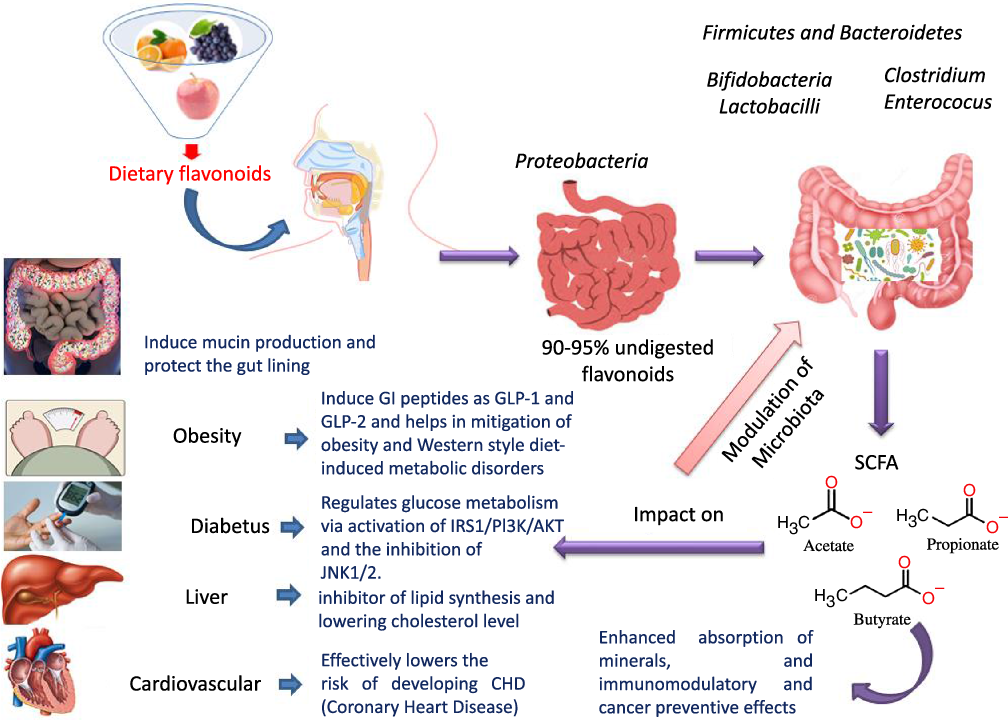
Fig. 3. Dietary flavonoids modulation of gut microbiota, action mechanisms and impact on health.
The two-way interaction between flavonoids and intestinal microbiota can be used to modulate the composition and diversity of the intestinal microbiome(Reference Selma, Espin and Tomas-Barberan21,Reference Oteiza, Fraga and Mills29,Reference Tomás-Barberán, Selma and Espín30) . This reciprocal relationship between dietary flavonoids and gut microbiota often enhances the biological activity of flavonoids, since their bioactive metabolites may have greater biological action than their parent compounds(Reference Selma, Espin and Tomas-Barberan21,Reference Ozdal, Sela and Xiao31) .
Flavonoids interaction with the human microbiome
Flavonols
Occurrence and metabolism
Flavonols are composed of a 3-hydroxyflavone base that is hydroxylated at certain positions (Fig. 2)(Reference Panche, Diwan and Chandra32). Flavonols are distributed in several foods, particularly onions, broccoli, tea, apples, tomatoes, grapes, berries, red wine, some fruit varieties and vegetables(Reference Panche, Diwan and Chandra32,Reference Hollman and Katan33) . The most commonly known flavonols include kaempferol, quercetin, myricetin and fisetin(Reference Iwashina34).
Table 1. Effect of different groups of individual flavonoids and flavonoid extracts on gut microbiota composition and health effects
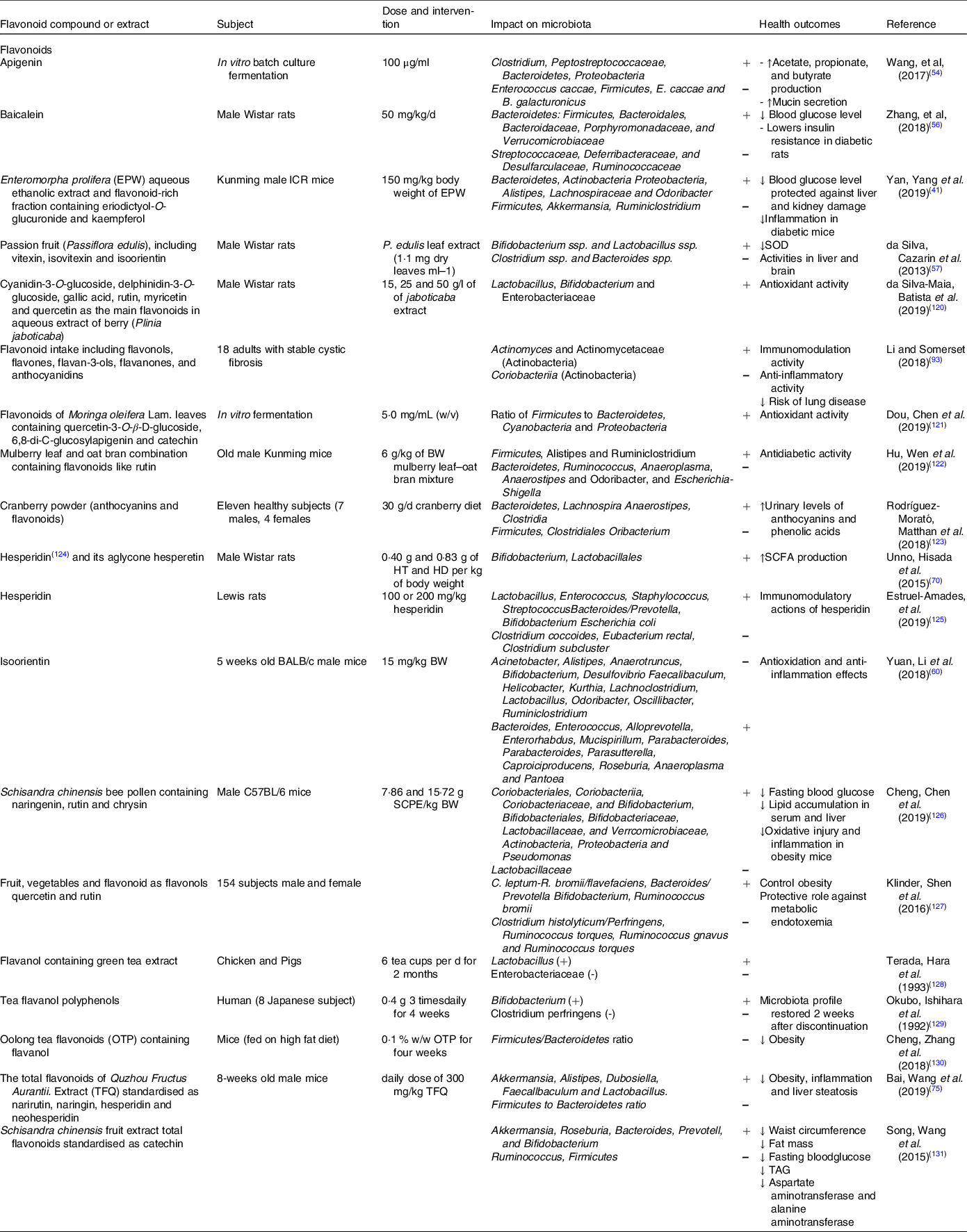
SOD, superoxide dismutase.
Flavonol glycosides are hydrolysed by microbial β-glucosidases to the corresponding aglycones which are then biotransformed by intestinal microbiota through mostly oxidative C-ring cleavage and demethylation, or dehydroxylation reactions to yield simple phenols and benzoates, see Fig. 4. For example, quercetin yields phloroglucinol, dihydrocaffeic acid, 2-(3,4-dihydroxyphenyl)acetic acid, 2-(3-hydroxyphenyl)acetic acid, 3,4-dihydroxybenzoic acid and 3-(3-hydroxyphenyl)propionic acid as major metabolites (Fig. 4). Other metabolites of flavonols include 2,4,6-trihydroxybenzoic acid, 3-(3,4-dihydroxyphenyl) benzoic acid methyl ester, vanillic acid and 3-(p-hydroxyphenyl) propionic acid. Additionally, myricetin which contains a trihydroxy ring-B yielded 2-(3-dihydroxyphenyl)acetic acid, 2-(3-hydroxyphenyl) acetic acid and 2-(3,4,5-trihydroxyphenyl) acetic acid. Whereas in the case of kaempferol (bearing a mono hydroxylated ring B), only 2-(4-hydroxyphenyl) acetic acid was produced(Reference Rechner, Smith and Kuhnle35). Mono-glycosylated flavonols such as quercetin-3-O-β-D-glucopyranoside showed faster metabolic breakdown by gut bacteria than the more complex di- and trisaccharides(Reference Simons, Renouf and Hendrich36). Both the type of sugar and glycosidic bond (α- or β- and C- or O-glycoside) also affect flavonoids stability inside the colon(Reference Hein, Rose and van’t Slot37), with C-flavonoid glycosides showing slower metabolic rates than O-glycosides.
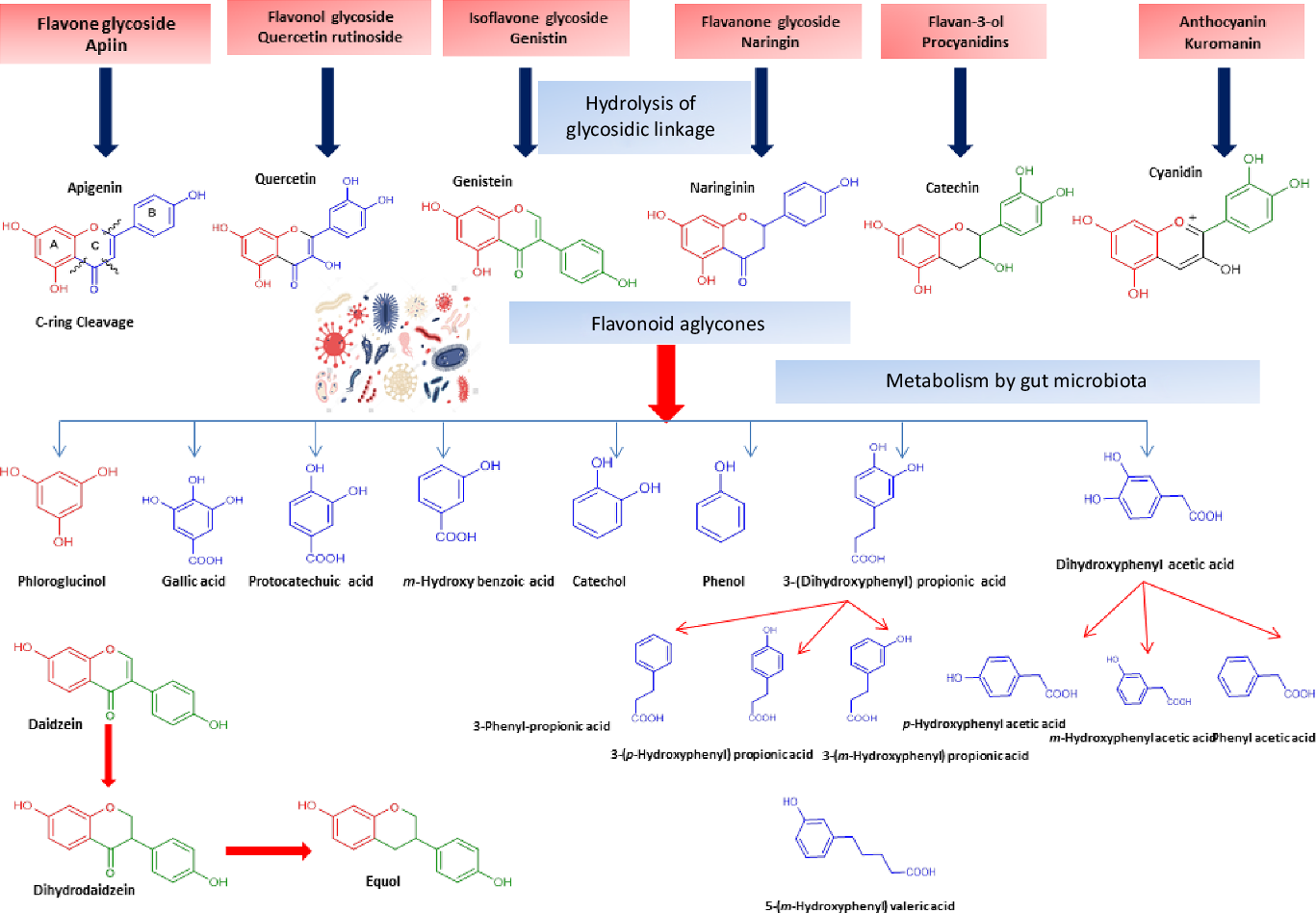
Fig. 4. Schematic representation of microbiota modulation by flavonoids and most common metabolites detected in colon.
Interactions with the microbial community
The impact of flavonol (or flavonol-rich extracts/foods) on the microbial community structure and diversity in the large intestine has been investigated in multiple studies (Table 1).
In vitro studies
The impact of flavonols on model microbial species has been extensively examined in vitro. The influence of the flavonol ‘rutin’ and its aglycone quercetin on the growth of the intestinal bacteria Enterococcus caccae, Bacteroides galacturonicus (DSM 3978), Lactobacillus sp. (DSM 20059), Ruminococcus gauvreauii (DSM 19829), Bifidobacterium catenulatum (DSM 16992) and E. coli (DSM 1116) was investigated at a dose of 20, 100 or 250 μg/ml of rutin and 4, 20 or 50 μg/ml of quercetin(Reference Duda-Chodak38). While quercetin inhibited the growth of all examined bacteria, especially Ruminococcus gauvreauii (with minimum inhibitory concentration (MIC) 20 μg/ml), Bacteroides galacturonicus and Lactobacillus (MIC 50 μg/ml) in a dose-dependent manner, no effect was observed in case of rutin. These results suggest that only flavonol aglycones can affect intestinal bacterial growth, such pattern was observed for other flavonoid glycosides such as flavanones, especially naringenin as described below(Reference Duda-Chodak38). Moreover, the metabolising capacity of Bifidobacterium and Lactobacillus on flavonoids and the anti-inflammatory potential of various metabolites on lipopolysaccharide-stimulated RAW264 macrophages was evaluated in vitro (Reference Kawabata, Sugiyama and Sakano39). Different flavonols were tested in the presence of B. adolescentis culture, with galangin, quercetin and fisetin to show significant inhibition of nitric oxide (NO) production induced by lipopolysaccharide and hence anti-inflammatory activity. Additionally, galangin prevented B. adolescentis growth by 30–70 % after 1–6 h co-culture, while quercetin and fisetin showed no effect on bacterial growth rate. On the other hand, biotransformation products of flavonols detected in the culture media, viz. 2–3)hydroxyphenyl)acetic acid, 2-(3,4-dihydroxyphenyl) acetic acid, 3-phenylpropionic acid, 3-(3,4-dihydroxyphenyl)propionic acid, phloroglucinol and resorcinol failed to suppress NO production(Reference Kawabata, Sugiyama and Sakano39). Accordingly, these results suggest that flavonols exerted weak modulatory activity against B. adolescentis, with only galangin to show inhibition capacity(Reference Kawabata, Sugiyama and Sakano39).
In vivo/animal studies
The impact of quercetin intake on the intestinal microbiota diversity in both fat- and carbohydrate-rich diets in rats was compared by Etxeberria et al. (2015). Quercetin consumption (30 mg/kg BW/d) was found to affect the gut microbiota composition by inhibiting the growth of bacterial species associated with diet-induced obesity such as Erysipelotrichaceae, Bacillus spp. and Eubacterium cylindroides, and to likewise modulate Firmicutes/Bacteroidetes ratio towards a higher Bacteroidetes content(Reference Etxeberria, Arias and Boqué40). In addition, quercetin prevented body weight gain, reduced serum insulin level, insulin resistance and reduced high-fat/carbohydrate-diet-induced gut microbiota dysbiosis(Reference Etxeberria, Arias and Boqué40). How the well-known antidiabetic potential of flavonols can be modulated by gut microbiota interaction has yet to be reported.
Additional studies investigated the antidiabetic potential of both Enteromorpha prolifera (green macroalgae) water–ethanol extract and its flavonoids-rich fraction enriched in kaempferol in diabetic mice(Reference Yan, Yang and Lin41). E. prolifera flavonols (150 mg/kg b.w.) significantly increased the relative abundance of members of the family Lachnospiraceae, as well as the genera Odoribacter, and Alisties. Notably, Alisties is one of the most abundant SCFA-producing bacteria(Reference Brown, Davis-Richardson and Giongo42). E. prolifera flavonols significantly increased Bacteroidetes, Actinobacteria and Proteobacteria populations, concurrent with decreased Firmicutes, Ruminiclostridium and Akkermansia (Reference Yan, Yang and Lin41). The same study also reported that E. prolifera flavonols could lower fasting blood glucose level, enhance oral glucose tolerance and prevent liver and kidney damages modulated by its anti-inflammatory action in diabetic mice. Whether biotransformed flavonols exert a more potential anti-inflammatory action inside the colon ought to be examined post their characterisation.
The potential of flavonols for alleviating systemic lupus erythematosus symptoms and severity was investigated(Reference Cuervo, Hevia and López43). Twenty women subjects suffering from systemic lupus erythematosus were provided with an orange-rich and apple-rich diet standardised to contain 300 mg·d−1 of flavonols. The observed increase in the relative abundance of Lactobacillus and Bifidobacterium populations in systemic lupus erythematosus patients was associated with the flavonol-rich diet. Enhancement of Bifidobacterium growth was suggested to benefit systemic lupus erythematosus patients due to the immunomodulatory effect associated with the presence of this bacterial genus(Reference Konieczna, Akdis and Quigley44). Specifically, B. bifidum promotes the induction of regulatory T cells (T regs), expressing chemokine receptors and potentially favouring mucosal homoeostasis(Reference López Suárez, González Rodríguez and Gueimonde Fernández45,Reference López, González-Rodríguez and Sánchez46) . Likewise, enhancement of Lactobacillus growth have a beneficial effect for systemic lupus erythematosus patients by modulating the host immune response that leads to improvement of disease symptoms and or severity(Reference Daly, Darby and Hall47,Reference Atarashi, Tanoue and Shima48) .
Various mechanisms have been suggested for the interaction of flavonols with intestinal microbiota(Reference Cardona, Andrés-Lacueva and Tulipani49). Mechanisms for inhibiting microbial growth could include interference with bacterial cell membrane structure to inhibit growth, modulate growth factors or suppress bacterial nucleic acid biosynthesis(Reference Kawabata, Sugiyama and Sakano39). However, such effects do not appear to be universal, since flavonols intake showed negative association with certain bacteria like Actinobacteria and Bifidibacteria, but positive association with others, for example, Blautia (Reference Etxeberria, Arias and Boqué40).
Flavones
Occurrence and metabolism
Flavones are a subgroup of flavonoids characterised by possessing a basic 2-phenyl-benzopyrone nucleus and the absence of hydroxylation at C-3 of ring C (Fig. 2)(Reference Panche, Diwan and Chandra32). Flavones are abundant as O-glycosidic forms in various dietary sources, for example, celery, parsley, red peppers, chamomile, mint and Ginkgo biloba (Reference Selma, Espin and Tomas-Barberan21). Citrus fruits and their peel are the richest source of polymethoxylated flavones such as tageretin, nobiletin and sinensetin(Reference Manach, Scalbert and Morand18). In addition, many of the C-glycosylated flavones are widespread in cereal crops(Reference Xiao50). Generally, O-glycosides are metabolised to their aglycones in the small intestine by human enzymes such as lactase phlorizin hydrolase and β-glucosidase(Reference Xiao and Hogger51). In contrast, C-glycosides reach the colon almost intact(Reference Zhang, Tie and Bao52). Flavones are metabolised by the action of colonic bacteria via C-ring degradation to yield phloretin chalcone, 3-(3,4-dihydroxyphenyl)-propionic acid, 3-(4-hydroxyphenyl)-propionic acid, 3-(3-hydroxyphenyl)-propionic acid and 4-hydroxycinnamic acid as major metabolites (Fig. 4)(Reference Hanske, Loh and Sczesny53).
Interactions with the microbial community
Several studies have examined the interaction between flavones and gut microbiota and the impact of such interaction on host’s physiology (Table 1).
In vitro studies
The modulatory activity of apigenin on pure cultures of Bacteroides galacturonicus, Bifidobacterium catenulatum, Lactobacillus rhamnosus and Enterococcus caccae, as well as on the gut microbiota from a donor’s faecal inoculum, was tested in vitro (Reference Wang, Firrman and Zhang54). Apigenin revealed an effective inhibition activity on both E. caccae and B. galacturonicus growth (100 µg/ml), while L. rhamnosus and B. catenulatum were not affected(Reference Wang, Firrman and Zhang54). Apigenin slightly increased the relative abundance of the Bacteroidetes from 0·14 % and 1·07 % in the control to 0·28 % and 1·95 % in apigenin-treated group at 4 h and 12 h, respectively. On the other hand, the relative abundance of Firmicutes decreased within a 48-h incubation period, resulting in an overall decrease in the Firmicutes/Bacteroidetes (F/B) population ratio. SCFA (acetate, propionate and butyrate) production also increased upon apigenin treatment(Reference Van Dorsten, Peters and Gross55) (Fig. 3). SCFA production may originate not only from the colonic microbial fermentation of carbohydrates in the culture medium, but rather from microbial metabolism of polyphenols though with the latter less in levels(Reference Blaut, Schoefer and Braune19).
In vivo/animal studies
The antidiabetic activity and gut microbiota modulation by baicalein (5,6,7-trihydroxyflavone) was examined in streptozotocin and high-fat-diet-induced diabetic rats(Reference Zhang, Sun and Yu56). The results revealed that the Firmicutes/Bacteroidetes (F/B) ratio significantly increased in the baicalein treatment group due to an increase in the relative abundance of members of the Bacteroidaceae and Porphyromonadaceae, concurrent with a decrease in the relative abundance of members of the families Streptococcaceae, Deferribacteraceae, Ruminococcaceae and Desulfarculaceae. Many of the taxa stimulated by baicalein treatment are SCFA producers, for example, members of the Bacteroides, Alloprevotella, Butyricimonas, Parabacteroides and Bacteroidales, and the family Prevotellaceae. Such results clearly indicate that the intestinal microbiome can mediate and enhance the antidiabetic action of flavonoids specially flavones, which thus can to be used as natural agent to support a type 2 diabetes preventive or antidiabetic lifestyle.
Another study investigated the antioxidant activity of flavone compounds from passion fruits and leaves (Passiflora edulis, maracuja/maracuya) water extract in vivo (Reference da Silva, Cazarin and Colomeu57). The antioxidant capacity of such compounds was inferred from a decrease in thiobarbituric acid reactive in the liver by 20 %, increased GSH content in kidneys by 40 %, a two-fold increase of glutathione reductase level, 3·5 times decreased glutathione peroxidase (GPx) abundance in liver, and a 45 % reduction of superoxide dismutase in liver and brain after treatment with P. edulis relative to the control group. Additionally, three flavones were found to be abundant in P. edulis leaf aqueous extract, identified as vitexin, isovitexin and isoorientin, which led to an increase of bacterial count in feces, especially of Bifidobacterium, Lactobacillus and total aerobic bacteria. Such a pattern was also observed in a study where rats fed with a diet rich in grape fibres, showing eventually higher levels of Lactobacillus and Bacteroides (Reference Pozuelo, Agis-Torres and Hervert-Hernández58). Interestingly, SCFA levels were reduced in groups treated with P. edulis leaf extract enriched in flavones, with a significantly lower percentage of acetic and butyric acids (21 and 66 %, respectively). These results contradict the effect of apigenin reported in ref(Reference Wang, Firrman and Zhang54) and could indicate differential effects of various flavones on bacterial growth depending on the nature of the administered flavones, although a mechanistic understanding of this process is currently unclear and other options exist, for example,, counteracting effects of other components of the maracuja extract(Reference Macfarlane and Macfarlane59).
Finally, the effects of the flavone isoorientin (luteolin 6-C-glucoside) on the intestinal microbiota and antioxidation, anti-inflammation and antibiosis of BALB/c mice were investigated in vivo (Reference Yuan, Li and He60). Isoorientin consumption was showed to promote body weight gain and increase the digestibility of crude proteins and the antioxidation capacity in mice. Additionally, isoorientin was shown to inhibit the growth of several bacterial genera such as Acinetobacter, Anaerotruncus, Bifidobacterium, Desulfovibrio, Faecalibaculum, Kurthia, Lachnoclostridium, Lactobacillus, Odoribacter and Ruminiclostridium. Moreover, isoorientin inhibited the growth of some inflammation-causing pathogenic bacterial genera such as Alistipes, Helicobacter and Oscillibacter (Reference Yuan, Li and He60).
Flavanones
Occurrence and metabolism
Flavanones are an important flavonoid subclass that is widely distributed in all citrus fruits such as orange, grapefruit, lime and lemon(Reference Panche, Diwan and Chandra32). Flavanones possess a 2,3-dihydro-2-phenylchromen-4-one structure, with a saturated C2–C3 bond (Fig. 2)(Reference Iwashina34). Hesperitin, naringenin and eriodictyol are examples of these phenolics commonly encountered in citrus fruits(Reference Tomás-Barberán and Clifford61) and are responsible for their bitter taste and several health effects(Reference Tomás-Barberán and Clifford61,Reference Shakour, Fayek and Farag62) . Flavanones are mostly found as glycosides, either rutinosides (-rhamnosyl-α-1→6-glucosides) or neohesperidosides (-rhamnosyl-α-1→2-glucosides) at C-7 of ring A(Reference Shakour, Fayek and Farag62,Reference Robards, Li and Antolovich63) . The flavanone metabolism is similar to that of flavonol and other flavonoid degradation pathways (Fig. 4). For example, naringin (naringenin-7-O-rhamnoglucoside) as a flavanone member, if subjected to sugar hydrolysis yields naringenin as the aglycone part (Fig. 4)(Reference Selma, Espin and Tomas-Barberan21). The aglycone suffers further breakdown via C-ring fission to yield phloroglucinol and 3-(3,4-dihydroxyphenyl) propionic acid as a major metabolite which can be further dehydroxylated to produce 3-(m-hydroxyphenyl) propionic acid (Fig. 4). Isoxanthohumol a major prenylflavonoid of hops (Humulus lupulus L.) is biotransformed by intestinal bacteria Eubacterium limosum via demethylation and formation of a potent bioavailable phyto-oestrogen 8-prenylnaringenin(Reference Possemiers, Heyerick and Robbens64,Reference Possemiers, Verstraete and Van de Wiele65) .
Interaction with the microbial community
Compared with flavonols and flavones, intact flavanones have a higher bioavailability in the human gut, due to the fact that a lower percentage is converted by intestinal microbiota(Reference Marín, Miguélez and Villar66). Therefore, a higher concentration reaches the distal colon and is absorbed by enterocyte cells(Reference Marín, Miguélez and Villar66).
In vitro studies
The effect of flavanones on microbial growth was also investigated in multiple in vitro studies. For instance, the effect of naringenin on the growth of a probiotic (Lactobacillus rhamnosus), a commensal (Escherichia coli) and two pathogenic bacteria (Staphylococcus aureus and Salmonella typhimurium) was studied in vitro (Reference Parkar, Stevenson and Skinner67). Naringenin enhanced the growth of L. rhamnosus and E. coli and inhibited the pathogens S. aureus (MIC 62·5 µg/ml), and S. typhimurium (MIC 125 µg/ml). Moreover, the growth inhibition effect on H. pylori by citrus flavanones, viz. hesperetin, naringenin and poncirin has been tested in vitro. Among the tested compounds, ponciretin was the most potent one and its MIC was 10–20 µg/ml(Reference Bae, Han and Kim68). In another study(Reference Duda-Chodak38), the effect of flavanones, viz. naringenin, naringin, hesperetin and hesperidin on 6-bacteria, that is, Bacteroides galacturonicus, Lactobacillus sp., E. caccae, Bifidobacterium catenulatum, Ruminococcus gauvreauii and E. coli was studied, revealing growth inhibition of all monitored species with interestingly aglycones to show higher activity compared with the corresponding glycosides(Reference Duda-Chodak38). Such strong inhibitory effect of flavonone aglycones might cause unfavourable changes in the composition of physiological microbiota in the human intestine(Reference Duda-Chodak38).
The impact of the flavanones naringenin and hesperidin on Bifidobaterium bifidum and B. adolescentis growth was also tested in vitro (Reference Gwiazdowska, Juś and Jasnowska-Małecka69). Results revealed inhibitory growth effects of hesperidin on the both Bifidobacterium species, whereby high doses (100 μg/ml) reduced the growth of B. bifidum and B. adolescentis by 20 and 50 %, respectively. On the other hand, naringenin (20 and 100 μg/ml) showed a 20 % increase in growth of B. bifidum compared with control cultures(Reference Gwiazdowska, Juś and Jasnowska-Małecka69).
In vivo/animal studies
The influence of citrus flavanones including hesperidin and its aglycone hesperetin on the composition, diversity and activity of gut microbiota was studied in vivo in rat models(Reference Unno, Hisada and Takahashi70). The hesperetin-rich diet increased the relative abundance of Bifidobacterium and Lactobacillus and decreased Clostridium subcluster XIVa population in feces. Notably, the Clostridium cluster XIVa proportion was increased by feeding a high-fat diet, whereas vegetarians with a fibre-rich diet had a low relative abundance of Clostridium cluster XIVa, compared with omnivores(Reference Kabeerdoss, Devi and Mary71,Reference Yoshimoto, Loo and Atarashi72) . Accordingly, alteration of the microbial composition by hesperetin and its derived or induced SCFA resulted in a significant decrease in abdominal adipose tissue accumulation, fatty acid synthesis, fatty acid oxidation and increased lipolysis in the body(Reference Den Besten, van Eunen and Groen73). Further, rats fed with a daily dose of hesperetin and hesperidin (0·40 g and 0·83 g per kg body weight) had a lower abdominal adipose tissue weight compared with those fed with the control diet. The hesperetin-rich diet showed a significant elevation in caecum content weigh,; whereas hesperidin (corresponding glycoside) fed groups recorded no change when compared with the control group. Hesperetin also elevated SCFA production in the intestine as a result of blocking the action of pancreatic α-amylase which led to an increase in fermentation by gut microbiota in the colon (Fig. 3)(Reference Gibson and Roberfroid74).
In another study, the impact of flavanones from Quzhou Fructus Aurantii (a dried unripe fruit of Rutaceae Citrus changshan-huyou) extract standardised for narirutin, naringin, hesperidin and neohesperidin on gut microbial diversity was studied in high-fat diet fed mice(Reference Bai, Wang and Wang75). Results revealed that Fructus Aurantii extract significantly reduced body weight, intestinal inflammation and liver steatosis in obese mice. Additionally, Fructus Aurantii flavanones extracts decreased the F:B ratio, increased the relative abundance of the genera Akkermansia and Alistipes, and decreased the relative abundance of the genera Dubosiella, Faecalibaculum and Lactobacillus (Reference Bai, Wang and Wang75).
Isoflavones
Occurrence and metabolism
Isoflavones exhibit a planar basic ring system with attachment of a benzene ring B at C-3, which differentiate their structure from other flavonoids (Fig. 2). A unique aspect of some isoflavonoids is their ability to bind to oestrogen receptors(Reference Panche, Diwan and Chandra32), hence their classification as natural phyto-oestrogens(Reference Yuan, Wang and Liu76,Reference Cornwell, Cohick and Raskin77) . Soya is a rich source of isoflavonoids(Reference Yuan, Wang and Liu76), with genistein, daidzein and glycitein as the major forms. Isoflavones such as genistein and daidzein are commonly utilised as selective oestrogen receptor modulators because of their mild oestrogenic activity in vivo (Reference Szkudelska and Nogowski78).
Isoflavones usually pass to the colon unabsorbed. Their bioavailability depends on the hydrolysis of glycosides into their bioactive aglycones such as, daidzein, genistein and glycitein via the action of β-glucosidase from gut microbiota(Reference Setchell, Brown and Zimmer-Nechemias79). Isoflavone aglycones are absorbed completely and taken up into the blood stream(Reference Linford and Dorsa80,Reference Zubik and Meydani81) The soya isoflavones were metabolised by the effect of some intestinal bacteria, such as Eggerthella spp., Enterococcus faecium, Adlercreutzia equolifaciens, Slackia equolifaciens, Lactobacillus mucosae, Bifidobacterium spp. and Bacteroides ovatu (Reference Duda-Chodak38). For example, genistein is metabolised to p-ethylphenol and 4-hydroxyphenyl-2-propionic acid, whereas daidzein is further reduced to O-demethylangolensin and equol(Reference Yuan, Wang and Liu76). Also, the metabolism of daidzein by faecal bacteria from four human individuals were identified as Lactobacillus mucosae EPI2, Enterococcus faecium EPI1, Veillonella sp and Finegoldia magna EPI3 was investigated. Dihydrodaidzein, O-desmethylangolensi, and equol were detected as major metabolites (Fig. 4)(Reference Decroos, Vanhemmens and Cattoir82). Due to its nonplanar structure, equol has a good bioavailability and is able to inhibit oxidative damage in situ. However, with the high bioavailability of equol, it should be noted that the biotransformation capacity of isoflavones to form equol in humans is much lower than that of animals(Reference Yuan, Wang and Liu76,Reference Setchell, Brown and Zimmer-Nechemias79) . Additionally, dihydrodaidzein, tetrahydrodaidzein, hydroxydaidzein, 6-hydroxydaidzein, 8-hydroxydaidzein, hydroxyphenylbenzopyran-4,7-diol and 2-dehydro-O-DMA have also been reported to be microbial metabolites of daidzein(Reference Yuan, Wang and Liu76).
Interactions with the microbial community
Almost all isoflavones, for example, daidzein, genistein and formononetin, exist as glucosides and are subjected to hydrolysis by intestinal β-glucosidases from gut microbiota to yield their corresponding more bioavailable aglycones(Reference Setchell, Brown and Zimmer-Nechemias79).
The inhibitory effect of genistein and daidzein against the gram-positive S. aureus, S. pasteurianus and B. cereus, as well as the gram-negative bacteria H. pylori and E. coli was tested in vitro (Reference Verdrengh, Collins and Bergin83). And 100-μM Genistein inhibited growth of all tested gram-positive spp., while in gram-negative only the growth of H. pylori was inhibited and E. coli growth was not affected. Compared with the structurally related daidzein, genistein was more active due to its ability to inhibit DNA topoisomerase II and tyrosine kinases enzymes(Reference Verdrengh, Collins and Bergin83). These results support the ability of the isoflavone genistein to inhibit the growth of pathogenic bacteria in the human gut. Additionally, the intestinal metabolism of daidzein by gut microflora yields equol a potent phyto-oestrogen that also exhibit anti-androgenic, anticancer, antioxidant and anti-inflammatory activities(Reference Kang, Lee and Rogozin84–Reference Qin, Shi and Liu86). Calycosin-7-O-β-D-glucoside, a glycosylated isoflavone, was shown to promote the growth of beneficial microbiota, viz. Lactobacillus and Bifidobacterium after oral administration to rats (40 mg/kg) which induced its angiogenic effect(Reference Ruan, Li and Li87)
Flavan-3-ols or catechins
Occurrence and metabolism
Flavan-3-ols, which commonly feature catechol moieties, include a wide variety of compounds such as catechin, epigallocatechin and epicatechin(Reference Panche, Diwan and Chandra32). Flavan-3-ol structures are characterised by having a B ring attached to C-2, with an absent carbonyl group at C-4 and double bonds between C-2 and C-3(Reference Hackman, Polagruto and Zhu88). They are abundant in fruits, tea and wine and are considered the primary supply of dietary phenolics. Green tea is considered to be the richest source of catchins as it contains about 30–42 % catechin of total phenols. Major tea catechins include (–)-epigallocatechin gallate which is considered the most abundant one with a share of 50–80 % of the total catchin concentration, along with other catchins such as (–)-epigallocatechin, (–)-epicatechin gallate and (–)-epicatechin(Reference Hackman, Polagruto and Zhu88,Reference Musial, Kuban-Jankowska and Gorska-Ponikowska89) . Catechins are metabolised in the colon by the action of intestinal microbiota to yield low molecular weight metabolites which may be absorbed or otherwise are excreted in feces(Reference Takagaki and Nanjo90). Interestingly, epicatechin when incubated with intestinal bacterial strains in vitro yields pyrogallol, 5-(3,4-dihydroxyphenyl) valeric acid, 5-(3-hydroxyphenyl) valeric acid, 3-(3-methoxyphenyl) valeric acid, 3-(3,4-dihydroxyphenyl) propionic acid, 3-(3-hydroxyphenyl)propionic acid and 2,3-dihydroxyphenoxyl 3-(3,4-dihydroxyphenyl) propionic acid as major metabolites(Reference Meselhy, Nakamura and Hattori91). The production of phenylpropionic acids or p-hydroxyphenyl acetic acids from flavan-3-ols and procyanidins is a result of C-ring cleavages (Fig. 4). Moreover, the inter individual variations in the human intestinal microbial metabolism was found to affect the metabolism of polyphenolics(Reference Liu, Vervoort and Beekmann6). An in vitro study was conducted to quantify the effect of inter individual variations in gut microbiota on the metabolism (−)-epicatechin by using faecal inoculum from twenty-four healthy donors. Two key metabolites were detected 1-(3′,4′-dihydroxyphenyl)-3-(2″,4″,6″-dihydroxyphenyl)-2-propanol (3,4-diHPP-2-ol) and 5-(3′,4′-dihydroxyphenyl)-γ-valerolactone (3,4-diHPV) which were to match in vivo metabolism. In addition, the use of such in vitro model could be further used to characterise in vivo colonic metabolism and related human plasma metabolites as well as to assess inter individual differences in the intestinal microbial metabolism of (−)-epicatechin(Reference Liu, Vervoort and Beekmann6). Another in vitro study was developed to characterise inter individual differences in gut microbial metabolism of (–)-epigallocatechin-3-O-gallate by using anaerobic human faecal incubations. The major metabolites were identified as gallic acid, pyrogallol, phenylpropane-2-ols, phenyl-γ-valerolactones and 5-(3′,5′-dihydroxyphenyl)valeric acid with substantial inter individual variations(Reference Liu, Vervoort and van den Elzen92). Moreover, the inter individual differences in intestinal microbial metabolism was found to affect not only (–)-epigallocatechin-3-O-gallate metabolism but rather its health effects(Reference Liu, Vervoort and van den Elzen92).
Interactions with the microbial community
Due to its poor bioavailability in the small intestine, large amounts of catechins come in contact with the colon’s microbiota(Reference Takagaki and Nanjo90). Tea flavonoids, especially catechins, have been shown to inhibit growth of pathogenic and opportunistic micro-organisms such as S. aureus, E. coli, S. typhimurium, Helicobacter pylori, Listeria monocytogenes and Pseudomonas aeruginosa (Reference Duda-Chodak, Tarko and Satora9).
The correlations between catechins intake and intestinal microbiota were studied in a group of cystic fibrosis patients(Reference Li and Somerset93). Results showed that gallocatechin consumption positively correlated with the relative abundance of members of the family Actinomycetaceae, specifically the genus Actinomcyes (phylum Actinobacteria), and negatively correlated with the family Coriobacteriaceae (Actinobacteria). Actinomcyes species were reported to play a role in pre-disposing or exacerbating cystic fibrosis and colorectal cancer symptoms. So, supplementation with nutritional recipes including catechin-rich diet could be potentially helpful for the management of cystic fibrosis and colorectal cancers(Reference Li and Somerset93).
Another interesting beneficial effect of grape seed extract and black raspberry catechins was demonstrated in ref(Reference Peng, Li and Green94), where the relative abundance of the butyrate producers Lachnospiraceae, Clostridiales and Ruminococcacceae increased in rats and human subjects subjected to a diet rich in oligomeric and polymeric proanthocyanidins. Higher butyrate levels have been shown to be associated withlower obesity(Reference Aravind, Wichienchot and Tsao4)(Fig. 5), improving bowel functions, preventing the transmission of pro-inflammatory molecules in systemic circulation and preventing metabolic endotoxemia(Reference Peng, Li and Green94). Butyrate also showed a direct effect on adipose tissue by enhancing adaptive thermogenesis in mice with depleted microbiota(Reference Li, Li and Li95).
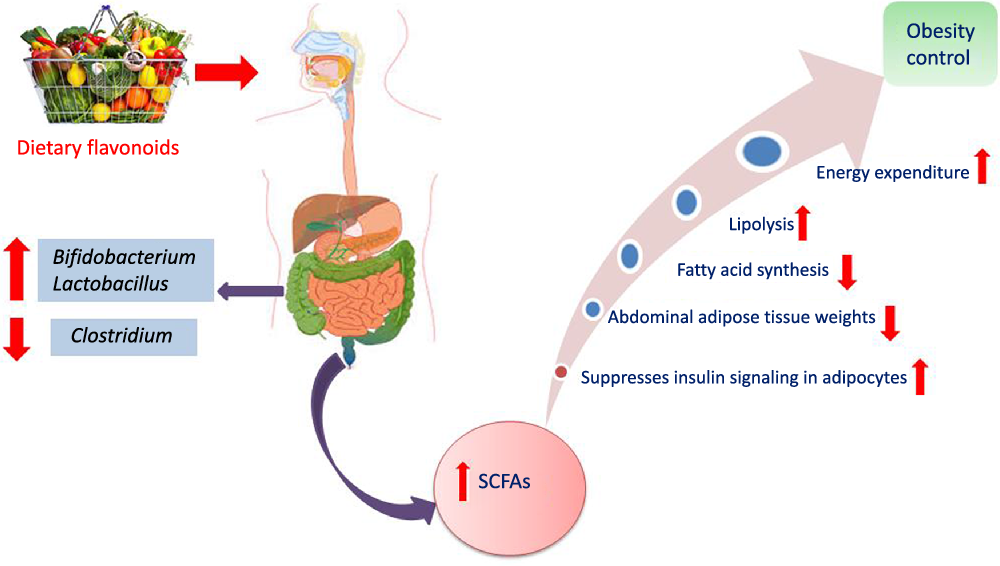
Fig. 5. Mechanism of obesity control by flavonoids mediated via modulation of gut microbiota.
Anthocyanins
Occurrence and metabolism
Anthocyanins are hydrophilic flavonoids that occur in coloured fruits and their peel, for example, cranberries, blueberries, black current, strawberries, red grapes and raspberries to name only a few, as well as in vegetables and grains including black rice, red rice and black soyabeans(Reference Sui, Zhang and Zhou96,Reference Wallace and Giusti97) . Anthocyanins are sensitive to degradation and natively occur usually as glycosides due to their higher stability(Reference Samadi, Bilsland and Georgakilas98). Cyanidin, delphinidin, malvidin and pelargonidin are the most commonly identified anthocyanins in different plant sources(Reference Matsumoto, Inaba and Kishi99). Although, antioxidant, anti-inflammatory, anti-carcinogenic and cardioprotective activities have been reported for anthocyanins(Reference Wang, Nair and Strasburg100,Reference Hou101) , their low bioavailability limits such effects(Reference Xiao and Hogger102). Anthocyanins are metabolised and biotransformed by gut microbiota in the human intestine(Reference Hanske, Engst and Loh103). For example, cyanidin 3-rutinoside is partially hydrolysed to its related glucoside and then is subsequently hydrolysed to yield the aglycone(Reference Keppler and Humpf104). The aglycones of anthocyanins are chemically unstable and undergo additional metabolism by the intestinal bacteria to yield small phenolic acids such as protocatechuic acid (3,4-dihydroxybenzoic acid) (Fig. 4)(Reference Aura105,Reference Vitaglione, Donnarumma and Napolitano106) . Other bacteria-generated anthocyanin metabolites include syringic acid, vanillic acid, phloroglucinol aldehyde, phloroglucinol acid, gallic acid and 3-O-methylgallic acid, depending on the anthocyanins precursor(Reference Keppler and Humpf104,Reference Forester and Waterhouse107) . The metabolic fate of (–)-epicatechin, procyanidin B1 and polymeric procyanidin was studied in vivo in humans(Reference Wiese, Esatbeyoglu and Winterhalter108). Blood plasma, urine or feces were collected from seven non-smoking men receiving 1 mg/kg body weight epicatechin and 2 mg/kg body weight procyanidin and polymeric procyanidin. Several in vitro identified gut-mediated catabolites were detected in feces including 3-hydroxybenzoic acid, 4-hydroxybenzoic acid, protocatechuic acid, vanillic acid, 3-hydroxyphenyl acetic acid, 4-hydroxyphenyl acetic acid, 3,4-dihydroxyphenyl acetic acid, 3-hydroxyphenyl propanoic acid, 4-hydroxyphenyl propanoic acid, 3,4-dihydroxyphenyl propanoic acid, 4 -hydroxy-5-(3′,4′-dihydroxyphenyl)valeric acid and 5-(3′,4′-dihydroxyphenyl)-valerolactone suggestive that in vivo model results parallel that reported from in vitro assays of microbial action(Reference Wiese, Esatbeyoglu and Winterhalter108).
Interactions with the microbial community and health outcomes
Multiple studies have revealed that anthocyanins and their metabolites could induce specific changes in human gut microbiota(Reference Faria, Fernandes and Norberto28). The daily consumption of anthocyanins in human diet has been estimated at 215 mg/d, with some studies to suggest for an average consumption of 57 − 69 mg/d(Reference Hidalgo, Oruna-Concha and Kolida109).
In vitro studies
In vitro incubation of malvidin-3-glucoside with faecal slurry enhanced total bacterial growth, including Bifidobaterium spp. and Lactobacillus spp.(Reference Hidalgo, Oruna-Concha and Kolida109), though without change in Bacteroides spp. growth. Moreover, malvidin-3-glucoside yielded a synergistic growth promotion effect for Bifidobaterium spp. and Lactobacillus spp. when mixed with other anthocyanins(Reference Hidalgo, Oruna-Concha and Kolida109). In addition, an increase in the growth of C. coccoides−Eubacterium rectale, a butyrate production group, was observed after incubation with malvidin-3-glucoside with such shifts in microbial community to present potential benefits to the host(Reference Hidalgo, Oruna-Concha and Kolida109). Moreover, anthocyanins-derived gallic acid (Fig. 4) reduced the growth of the potentially harmful Clostridium histolyticum, with no significant effect on beneficial bacteria populations(Reference Hidalgo, Oruna-Concha and Kolida109). Also, gallic acid significantly decreased Bacteroides spp. growth and enhanced Atopobium spp.(Reference Hidalgo, Oruna-Concha and Kolida109).
In vivo/animal studies
As described above, anthocyanins are not absorbed in the stomach or small intestine and are metabolised by the colon microbiota. Sánchez-Patán et al. observed a decreased level of Clostridium histolyticum in human faecal samples incubated with red wine extract, standardised to the anthocyanins malvidin-3-O-glucoside, peonidin and petunidin-3-O-glucosides(Reference Sánchez-Patán, Cueva and Monagas110). Such studies were also confirmed in vivo in a study using red wine consumption by human volunteers(Reference Queipo-Ortuño, Boto-Ordóñez and Murri111). Another study examined the gut microbiota composition in human volunteers after red wine and dealcoholised red wine consumption. Results showed an increase in relative abundance of Firmicutes and a lower F:B ratio after red wine intake(Reference Queipo-Ortuño, Boto-Ordóñez and Murri111). Additionally, Bacteroides and Prevotellaceae abundance was lower than those post-anthocyanin consumption periods, whereas the Clostridium abundance was high(Reference Queipo-Ortuño, Boto-Ordóñez and Murri111). In addition to changes in microbial composition, a significant decrease in blood pressure and serum TAG, total cholesterol, HDL-cholesterol and C-reactive protein was observed as a consequence of red wine consumption both dealcoholised or not. In another study, 6 weeks ingestion of a prebiotic anthocyanidin-rich wild blueberry drink led to a significant elevation of Lactobacillus acidophilus and Bifidobacterium spp. populations(Reference Vendrame, Guglielmetti and Riso112). Finally, anthocyanin-derived gallic acid was shown to reduce the growth of potentially harmful pathogenic bacteria, such as Clostridium histolyticum and Bacteroides spp., without any negative effect on the monitored beneficial bacteria(Reference Parkar, Trower and Stevenson113).
In another study, supplementation of mice diet with a polyphenol-rich source such as apples resulted in a significant elevation in Lactobacillus spp. and Bifidobacterium spp.(Reference Espley, Butts and Laing114). In a third study, 6 weeks of blueberry (Vaccinium angustifolium) drink consumption enriched with the anthocyanins peonidin glucose, malvidin galactose, delphinidin glucose and delphinidin galactose by human volunteers significantly elevated Bifidobacterium spp. and Lactobacillus spp.(Reference Vendrame, Guglielmetti and Riso112,Reference Molan, Lila and Mawson115) .
The role of habitual anthocyanin intake and association with gut microbiome modulation and visceral abdominal fat accumulation was studied in ref(Reference Jennings, Koch and Jensen116). Collectively, a higher anthocyanin intake was associated with higher microbial diversity and abundance of Firmicutes, Clostridiales, Ruminococcaceae and Roseburia (Reference Jennings, Koch and Jensen116). The consumption of anthocyanin-rich foods was associated with a decrease in visceral abdominal adipose tissue, high levels of Clostridiales and Ruminococcaceae and lower concentrations of Clostridium XIVa. Such anthocyanin activity is mediated via their microbiotically derived metabolites. These are present in the blood stream in significantly higher levels and for a longer time than the unaltered anthocyanins. These catabolites provide more valuable vascular and anti-inflammatory activities than the metabolites produced and absorbed in the small intestine(Reference Czank, Cassidy and Zhang117,Reference Amin, Czank and Raheem118) .
Collectively, the above studies present the anthocyanins as a valuable health promotors via modulation of commensal gut microbiota(Reference Parkar, Trower and Stevenson113), as well as by exhibiting a direct impact through the biological activities of them and from them generated metabolites. Anthocyanin-rich foods hence can be considered valuable prebiotic modulators that can change the gut community towards higher levels of Lactobacillus spp. and Bifidobacterium spp.(Reference Bialonska, Ramnani and Kasimsetty119)
Conclusion and future perspectives
The human diet is readily modifiable variable that can significantly impact the consumers’ health, either directly or indirectly via metabolic activity of the host’s microbiota action or by alteration of the microbiota composition. A balanced gut microbiota modulates appetite regulation, energy management, obesity, diabetes, immune function, allergy, behavioural disturbance, heart disease and colon cancer. Here, we highlighted the complex interactions between flavonoid intake and the gut microbiota. This review highlights the overall, mostly positive, impacts of flavonoid consumption through enriching potentially beneficial members of the gut microbioata, for example, Bifidobacterium and Lactobacillus, often occurring at the expense of Clostridium spp. We also highlighted other potential benefits associated with flavonoid consumption such as the production of breakdown products believed beneficial to human intestinal cells such as SCFA, that is, acetate, propionate and butyrate. Although our understanding of the correlation between flavonoid consumption, human gut microbiota and biological activities is rapidly expanding, additional research on documenting the impact of various specific flavonoid compounds, various doses and regiments on a wider range of chronic diseases are required. There is a need to identify the crucial drivers and those of the highest general relevance. Studies combining and correlating microbial community analyses not only with certain flavonoid or other dietary compounds intake with direct and accurate measurements of health outcomes are especially needed. A better reproducibility, understanding and assessment of results in such research, and the impact of ethnic, age, health and prevalent diet variations are needed. Only through such assessments, supplements, diet regimes, prebiotics and probiotics can be confidently suggested to fully exploit the health benefits of flavonoids on a wider scale.
Acknowledgements
M. A. F. acknowledges the funding received from the Alexander von Humboldt Foundation, Germany.
CRedit author contributions: M. H. B.: data collection curation and writing – original draft. M. E.: writing – review and editing. L. W.: review and editing. M. A. F.: supervision, conceptualisation, investigation, and writing – review and editing. All authors contribute to the revision of final manuscript.
The authors declare no conflict of interest.