Since it was first described by Ludwig( Reference Ludwig, Viggiano and McGill 1 ), non-alcoholic fatty liver disease (NAFLD) has progressed from a poorly understood liver disease to a more comprehensive one with better-defined boundaries. NAFLD is increasingly being diagnosed worldwide and is considered to be the most common liver disorder in Western countries, affecting at least a quarter of the general population( Reference Rector, Thyfault and Wei 2 , Reference Lazo and Clark 3 ). NAFLD encompasses a spectrum of disease states, from steatosis (fatty liver) and non-alcoholic steatohepatitis (steatosis with inflammatory changes) to fibrosis, cirrhosis and hepatocellular carcinoma( Reference Zafrani 4 ). Excess liver fat is believed to be a manifestation of the metabolic syndrome( Reference Marchesini and Babini 5 ), and, not surprisingly, non-alcoholic steatohepatitis has been found to be associated with obesity, insulin resistance, dyslipidaemia and type 2 diabetes in human subjects( Reference Anstee and Goldin 6 ). Most obese adults have hepatic steatosis, and at least one-third of these individuals will eventually develop worsening NAFLD( Reference Adams, Lymp and St Sauver 7 , Reference Browning, Szczepaniak and Dobbins 8 ). Therefore, the prevalence of NAFLD will probably rise with obesity rates.
Effective control of hepatic steatosis may improve insulin resistance and attenuate the progression to NAFLD or more serious complications. Weight loss and lower dietary fat intake are the cornerstones of treatment for steatosis, yet these interventions are marginally effective in the long term( Reference Clark 9 ). Bariatric surgery is effective for weight loss, but it is highly invasive, expensive and limited to selected volunteers who meet the rigorous screening criteria. Several drugs are under investigation for treating NAFLD, but the results are inconsistent. No drug has yet been approved by the Food and Drug Administration (FDA) for this purpose( Reference Lam and Younossi 10 ). Therefore, novel drugs or supplementary foods are urgently needed for the treatment of NAFLD.
Probiotic bacteria, most of which are classified as lactic acid bacteria including Lactobacillus sp., Bifidobacterium sp. and Enterococcus sp.( Reference Klein, Pack and Bonaparte 11 ), have become a promising alternative for the treatment of various diseases. Knowledge on the probiotic effects against diseases, such as diarrhoea, gastroenteritis and inflammatory bowel disease, cancer, allergy, and bacterial and viral infections, has been accumulated( Reference Parvez, Malik and Ah Kang 12 ). Moreover, recent studies( Reference Adams 13 – Reference Salminen, Ouwehand and Benno 15 ) have indicated that the viability of probiotic bacteria is indispensable for the probiotic effects. Previously, we showed that the administration of heat-treated Enterococcus faecalis FK-23 (FK-23), which was isolated from the faeces of a healthy human subject, exhibits an anti-obesity effect in high-fat diet (HFD)-induced mice( Reference Motonaga, Kondoh and Hayashi 16 ). This implies the possibility of attenuating hepatic steatosis by the administration of FK-23, since it has been reported that atherogenic dyslipidaemia after adjustment for obesity, physical activity and hyperglycaemia is associated with hepatic steatosis( Reference Makadia, Blaha and Keenan 17 ). Although there have been few reports( Reference Li, Yang and Lin 18 – Reference Zhao, Higashikawa and Noda 21 ) demonstrating the efficacy of probiotic administration on NAFLD, the detailed mechanisms are not yet known. In the present study, we examined whether the administration of heat-treated FK-23 attenuates HFD-induced hepatic steatosis in mice and enhances the mRNA expression levels of genes involved in fatty acid oxidation in the liver.
Materials and methods
Preparation of Enterococcus faecalis FK-23
FK-23 was prepared as described previously( Reference Motonaga, Kondoh and Hayashi 16 ). Briefly, FK-23 cells were cultured in a broth medium containing 2·46 % glucose, 1·4 % yeast extract, 0·77 % peptone and 4·39 % K2HPO4 for 18 h at 37°C, and the cells were collected by centrifugation. After washing with distilled water, the cells were heated at 110°C for 10 min. The cells were then lyophilised and used as FK-23.
Animal experiments
All animal experiments were performed according to the guidelines for the care and use of laboratory animals approved by Nichinichi Pharmaceutical Corporation Limited. Male C57BL/6J mice (6 weeks old) were purchased from Japan SLC. All animals were housed in cages with a 12 h light–12 h dark cycle. Temperature and humidity were controlled at 25·0 ± 1·0°C and 55·0 ± 5·0 %, respectively. After 1 week of acclimatisation, mice were divided into four groups (n 5 per group) and started on one of four treatments on day 0: standard diet (SD); standard diet supplemented with FK-23 (SD+FK); HFD; or HFD supplemented with FK-23 (HFD+FK). For 11 weeks, mice in the SD and SD+FK groups were given a SD with 10 % of energy as fat (diet D12450B, 16·11 kJ/g (3·85 kcal/g); Research Diets), and those in the HFD and HFD+FK groups were fed a HFD with 60 % of energy as fat (diet D12492, 21·93 kJ/g (5·24 kcal/g); Research Diets). FK-23 was supplemented in the drinking water at a concentration of 2 % (w/w) and administered to the SD+FK and HFD+FK groups. FK-23 was applied in cycles, each consisting of 9 d of FK-23 administration followed by a 5 d interval with normal drinking water. The cycles were repeated throughout the experimental period of 11 weeks (79 d). Body weight was measured once per week. After 79 d, mice were fasted for 24 h and killed. Blood samples were obtained from the orbital sinus, and all mice were killed by cervical dislocation. Epididymal fat pads and liver samples were collected and weighted. The liver tissue was fast fixed and lightly washed with saline. Then, a portion of the liver was placed in 10 % formalin fixation solution, and another portion was frozen in liquid N2 and stored at − 80°C until analysis. The mucosa of the small intestine was also collected and frozen under the same condition.
Blood biochemical analysis
Blood glucose was measured using an Accu-Chek glucometer (Roche Diagnostics GmbH), according to the manufacturer's recommendations. Blood supernatants were obtained as serum after centrifugation. Alanine aminotransferase, aspartate aminotransferase, total cholesterol, TAG, phospholipids and NEFA in serum were measured at Medic Corporation Limited.
Histopathological staining
The 10 % formalin-fixed liver tissue was embedded in paraffin. The fixed and embedded tissues were cut into 2–3 μm-thick coronal sections for haematoxylin–eosin staining. Histological assessment was performed using light microscopy at 100 × magnification. Histological examination was performed using the histological scoring system for steatosis( Reference Kleiner, Brunt and Van Natta 22 ) in a blinded manner. For evaluation of steatosis, three individuals independently and randomly selected five fields for each mouse. The values obtained were then averaged for each mouse.
Lipid extraction and lipid classification
Total lipid was extracted from the liver according to the Bligh and Dyer method( Reference Bligh and Dyer 23 ). The total lipid extract was dried under a gentle stream of N2 and dissolved in chloroform–methanol (2:1). Lipid classes were determined using Iatroscan MK-6 (Mitsubishi Chemical Medience Corporation) and quantified with the flame ionisation detector. The samples were applied to conditioned silica gel SIII chromarods (5 μm particle size) using a 1 μl disposable micropipette. Chromarods were developed in a glass tank. The solvent system used for lipid separation was hexane–diethyl ether–acetic acid (80:20:1). After development, the chromarods were dried and analysed using a flame ionisation detector. The flow rate of hydrogen gas was 160 ml/min, airflow rate was 1500 ml/min and the scanning speed was 30 s/scan. Iatrocorder TC-21 (Mitsubishi Chemical Medience Corporation) was used to record the chromatogram and individual peak areas. Lipid classes were identified by comparison of retention times with those of standards (16 : 0 cholesterol ester, 16 : 0 TAG, 16 : 0 NEFA, 16 : 0 diacylglycerol, cholesterol and phospholipid). The absolute amount of TAG in the liver was quantified using calibration curves. 16 : 0 TAG solutions with different concentrations were applied under the same condition, and then the peak area was calculated.
Gene expression analysis
Total RNA was extracted from the liver or the mucosa of the small intestine by the acid–guanidinium–phenol–chloroform method using Isogen (Nippon Gene). Real-time PCR was conducted using a 7300 Real-Time PCR System (Applied Biosystems), and the PCR was performed with a Power SYBR Green PCR Master Mix. PCR conditions were as follows: one cycle at 95°C for 10 min; forty cycles at 95°C for 15 s and 60°C for 1 min; one cycle at 95°C for 15 s, 60°C for 30 s and 95°C for 15 s. β-Actin was used as an internal control. The primer pairs used in the present study are listed in Table 1.
Table 1 Oligonucleotide primer sequences used in the present study
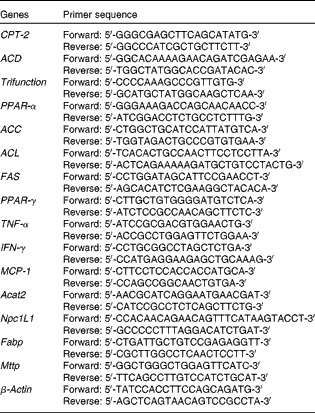
CPT-2, carnitine palmitoyltransferase-2; ACD, acyl-CoA dehydrogenase; Trifunction, trifunctional enzyme; ACC, acetyl-CoA carboxylase; ACL, ATP citrate lyase; FAS, fatty acid synthase; IFN-γ, interferon-γ; MCP-1, monocyte chemotactic and activating factor; Acat2, acetyl-CoA-acetyltransferase 2; Npc1L1, Niemann–Pick C1-like 1; Fabp, fatty acid-binding protein; Mttp, microsomal TAG transfer protein.
Statistical analysis
Data are expressed as means with their standard errors. The significance of the differences among the groups was determined by Fisher's protected least significant difference. P values of < 0·05 were considered statistically significant.
Results
Effects of Enterococcus faecalis FK-23 on the weights of total body, liver and epididymal fat pad
The changes in body weight, liver weight and epididymal fat pad weight are shown in Table 2. No difference was observed in either body weight or body-weight gain between the SD and SD+FK groups at the end of the feeding period. Compared with the SD group, mice in the HFD group had significantly larger body weights and greater body-weight gain. The HFD+FK group had a smaller increase in body weight than the HFD group; however, this difference was not statistically significant (P= 0·0569). The absolute liver weight of mice in the HFD group was significantly higher than those in the SD group; however, no significant difference was observed in the liver:body weight ratio between the SD and HFD groups. Compared with the HFD group, the increase in the liver:body weight ratio under the HFD condition was significantly (P< 0·05) inhibited by the treatment with FK-23. The absolute and relative epididymal fat weights of the HFD group were significantly higher than those of the SD group. FK-23 supplementation had no effect on the absolute or relative epididymal fat weight of mice fed a HFD.
Table 2 Body weight, liver weight and epididymal fat pad weight of mice (Mean values with their standard errors)
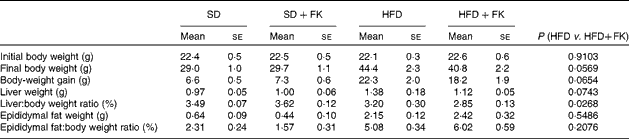
SD, standard diet; SD+FK, standard diet supplemented with Enterococcus faecalis FK-23; HFD, high-fat diet; HFD+FK, high-fat diet supplemented with Enterococcus faecalis FK-23.
Effects of Enterococcus faecalis FK-23 on serum biochemical parameters
Table 3 shows the biochemical parameters of blood obtained from mice fed a SD or HFD in the presence or absence of FK-23. After 11 weeks of feeding, mice in the HFD group had increased levels of blood glucose and alanine aminotransferase compared with those in the SD group. The administration of FK-23 significantly alleviated these effects. Mice in the HFD group showed significantly decreased levels of TAG and NEFA compared with those in the SD group. FK-23 supplementation had no effect on the levels of TAG and NEFA. There were no appreciable differences in aspartate aminotransferase, total cholesterol or phospholipid levels among all the groups.
Table 3 Biochemical parameters of blood (Mean values with their standard errors)
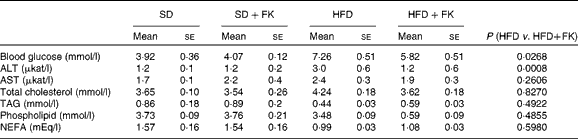
SD, standard diet; SD+FK, standard diet supplemented with Enterococcus faecalis FK-23; HFD, high-fat diet; HFD+FK, high-fat diet supplemented with Enterococcus faecalis FK-23; ALT, alanine aminotransferase; AST, aspartate aminotransferase.
Effects of Enterococcus faecalis FK-23 on histopathology of the liver
Changes in the degree of infiltration of lipid droplets in the liver were observed, as shown in Fig. 1(a)–(d). Although the liver histological sections of the SD and SD+FK groups were free of lipid droplets, increased accumulation of lipid droplets was observed in the HFD group after 11 weeks of treatment, leading to a condition of hepatic steatosis. The treatment with FK-23 ameliorated the increase in the accumulation of lipid droplets (Fig. 1(d)). In addition, the analysis of the histological scoring system for steatosis revealed significant differences between the HFD and HFD+FK groups (Fig. 1(e)).
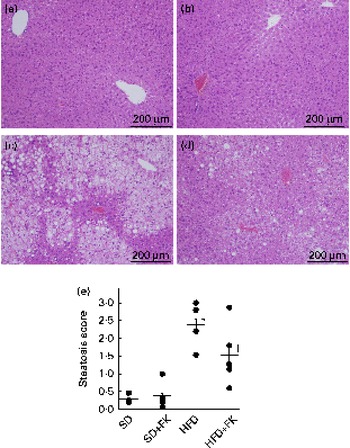
Fig. 1 Enterococcus faecalis FK-23 (FK-23) treatment prevents high-fat diet (HFD)-induced hepatic steatosis. (a)–(d) Changes in the degree of infiltration of lipid droplets in the liver were observed after haematoxylin and eosin staining of the liver tissue. Increased infiltration of lipid droplets was apparent in the liver tissue of (c) the HFD group compared with that of (a) the standard diet (SD) group, whereas decreased infiltration of lipid droplets was observed in (d) the HFD+FK (high-fat diet supplemented with Enterococcus faecalis FK-23) group when compared with the HFD group. No infiltration of lipid droplets was observed in the (a) SD and (b) SD+FK (standard diet supplemented with Enterococcus faecalis FK-23) groups. (e) For evaluation of steatosis, three individuals independently and randomly selected five fields for each mouse. Values are means, with their standard errors represented by bars. * Mean value was significantly different from that of the SD group (P< 0·05). † Mean value was significantly different from that of the HFD group (P< 0·05).
Effects of Enterococcus faecalis FK-23 on hepatic lipid profile
To gain insight into the mechanism of FK-23 against hepatic steatosis, the absolute amount of TAG was analysed, because the level of TAG has been reported to be correlated with the pathogenesis of NAFLD( Reference Kawano and Cohen 24 ). As shown in Fig. 2, the TAG level of hepatic lipid was increased after feeding the HFD; however, the increased TAG level was restored to the normal level in the HFD+FK group. Next, the lipid class was analysed after the extraction of total hepatic lipid from the liver tissue. The relative amounts of cholesterol ester, TAG, NEFA, cholesterol, diacylglycerol and phospholipid in total hepatic lipid are given in Table 4. Although statistically significant differences were not observed in the relative amounts of these lipids between the HFD and HFD+FK groups, the ratio of the TAG level tended to decrease in the HFD+FK group compared with the HFD group (Table 4).
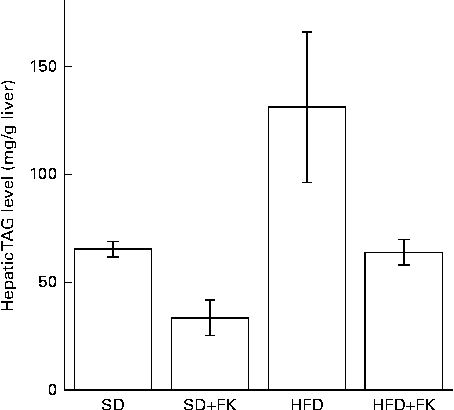
Fig. 2 Enterococcus faecalis FK-23 (FK-23) supplementation attenuates fat accumulation induced by high-fat diet (HFD) in the liver. Mice were fed with the standard diet (SD) or HFD in the presence or absence of FK-23 (2 %) in the drinking water. Values are means, with their standard errors represented by vertical bars. SD+FK, standard diet supplemented with Enterococcus faecalis FK-23; HFD+FK, high-fat diet supplemented with Enterococcus faecalis FK-23.
Table 4 Lipid classification in the liver (Mean values with their standard errors)
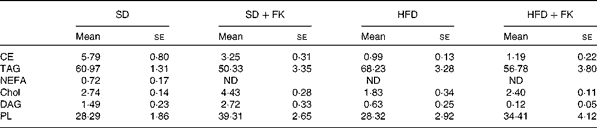
SD, standard diet; SD+FK, standard diet supplemented with Enterococcus faecalis FK-23; HFD, high-fat diet; HFD+FK, high-fat diet supplemented with Enterococcus faecalis FK-23; CE, cholesterol ester; ND, not detected; Chol, cholesterol; DAG, diacylglycerol; PL, phospholipid.
Effects of Enterococcus faecalis FK-23 on the expression levels of genes involved in lipid metabolism
The balance between lipid acquisition, such as fatty acid uptake and lipid synthesis, and mitochondrial fatty acid oxidation is one of the major factors that has an impact on TAG accumulation in hepatocytes( Reference Kawano and Cohen 24 ). First, to investigate whether the administration of FK-23 affects the uptake of fatty acid from the small intestine, the mRNA expression levels of acetyl-CoA-acetyltransferase 2 (Acat2), Niemann–Pick C1-like 1 (Npc1L1), fatty acid-binding protein (Fabp) and microsomal TAG transfer protein (Mttp) were analysed in the upper and lower parts of the small intestine. The analysis revealed that there was no significant difference in the expression levels of these genes between the HFD and HFD+FK groups (Fig. 3).
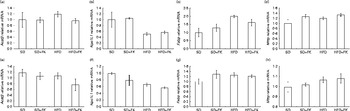
Fig. 3 mRNA expression levels in the (a)–(d) upper and (e)–(h) lower parts of the small intestine. Each gene was normalised to the mRNA expression level of β-actin. The mRNA expression level for the standard diet (sd) group was set at 1, and relative expression levels are expressed as fold induction for the SD+FK (standard diet supplemented with Enterococcus faecalis FK-23), HFD (high-fat diet) and HFD+FK (high-fat diet supplemented with Enterococcus faecalis FK-23) groups. Values are means, with their standard errors represented by vertical bars. Acat2, acetyl-CoA-acetyltransferase 2; Npc1L1, Niemann–Pick C1-like 1; Fabp, fatty acid-binding protein; Mttp, microsomal TAG transfer protein.
Next, to investigate whether the administration of FK-23 affects fatty acid oxidation and lipid synthesis, the mRNA expression levels were analysed in the liver. It is known that carnitine palmitoyltransferase-2 (CPT-2), acyl-CoA dehydrogenase (ACD), trifunctional enzyme (trifunction) and PPAR-α are involved in fatty acid oxidation, and that acetyl-CoA carboxylase (ACC), ATP citrate lyase (ACL) and fatty acid synthase (FAS) are involved in fatty acid synthesis( Reference Kawano and Cohen 24 ). Although the expression levels of CPT-2, ACD, trifunction and PPAR-α were lower in the HFD group than in the SD group (Fig. 4), the expression levels of CPT-2, trifunction and peroxisome proliferator-activated receptor (PPAR-α) were slightly higher in the HFD+FK group than in the HFD group (P= 0·1158, P= 0·1136 and P= 0·0807, respectively). The expression levels of ACC and FAS under the HFD condition were up-regulated by the administration of FK-23 (Fig. 4).
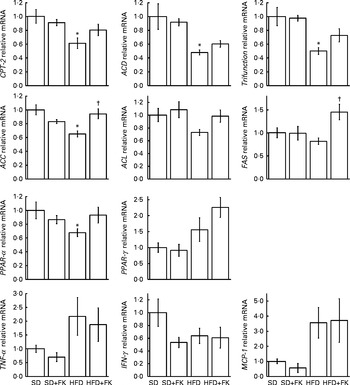
Fig. 4 mRNA expression levels in the liver. Each gene was normalised to the mRNA expression level of β-actin. The mRNA expression level for the standard diet (sd) group was set at 1, and relative expression levels are expressed as fold induction for the SD+FK (standard diet supplemented with Enterococcus faecalis FK-23), HFD (high-fat diet) and HFD+FK (high-fat diet supplemented with Enterococcus faecalis FK-23) groups. Values are means, with their standard errors represented by vertical bars. * Mean value was significantly different from that of the SD group (P< 0·05). † Mean value was significantly different from that of the HFD group (P< 0·05). CPT-2, carnitine palmitoyltransferase-2; ACD, acyl-CoA dehydrogenase; Trifunction, trifunctional enzyme; ACC, acetyl-CoA carboxylase; ACL, ATP citrate lyase; FAS, fatty acid synthase; IFN-γ, interferon-γ; MCP-1, monocyte chemotactic and activating factor.
Since chronic inflammation by feeding a HFD is known to be associated with lipid metabolism, we next analysed the expression levels of pro-inflammatory cytokines. The analysis revealed that there was no significant difference in the levels of TNF-α, interferon-γ (IFN-γ) and monocyte chemotactic and activating factor (MCP-1) between the HFD and HFD+FK groups (Fig. 4).
Discussion
Probiotics have been proposed to exert a variety of beneficial effects on health. Recent studies( Reference Adams 13 – Reference Salminen, Ouwehand and Benno 15 ) have indicated that probiotic effects are derived not only from live probiotics but also from dead probiotics. Previously, we showed that the administration of heat-treated FK-23 attenuates HFD-induced obesity in mice( Reference Motonaga, Kondoh and Hayashi 16 ). Therefore, in the present study, we focused on the possibility of attenuating hepatic steatosis, which is associated with atherogenic dyslipidaemia after adjusting for obesity, physical activity and hyperglycaemia( Reference Makadia, Blaha and Keenan 17 ), by the administration of FK-23.
In the present study, we used a mouse model of HFD-induced hepatic steatosis. The histological analysis of the liver section (Fig. 1) showed an intensive infiltration of lipid droplets into the cytoplasm of hepatocytes in HFD-fed mice compared with SD-fed mice. This result indicates the successful generation of the hepatic steatosis model in mice. Using this model, we examined whether oral administration of FK-23 attenuates HFD-induced hepatic steatosis. HFD-induced accumulation of lipid droplets in hepatocytes was significantly attenuated by the administration of FK-23 (Fig. 1). Since TAG is stored in lipid droplets within hepatocytes, the TAG level of hepatic lipid was analysed. Consistent with the histological findings, the administration of FK-23 tended to decrease the TAG level of hepatic lipid (Figs. 1 and 2 and Table 4). Furthermore, compared with the HFD group, the administration of FK-23 under the HFD condition tended to decrease body-weight gain and liver weight (Table 2), and significantly reduced the level of alanine aminotransferase in serum and blood glucose (Table 3). These results suggest that the administration of FK-23 attenuates HFD-induced hepatic steatosis.
The balance between the absorption and metabolism of lipid is correlated with the progression of hepatic steatosis( Reference Kawano and Cohen 24 ). However, there were no differences observed between the HFD and HFD+FK groups in relation to the expression levels of Acat2, Npc1L1, Fabp and Mttp genes that were involved in lipid absorption in intestinal epithelial cells (Fig. 3). This result suggests that the attenuation of HFD-induced hepatic steatosis by the administration of FK-23 was not associated with the absorption of lipid but with the metabolism of lipid.
PPAR-α regulates a large number of target genes involved in lipid metabolism including fatty acid β-oxidation pathways in the mitochondria and peroxisome( Reference Desvergne and Wahli 25 , Reference Gonzalez and Shah 26 ). In addition, PPAR-α-deficient mice have been shown to exhibit hepatic steatosis( Reference Hashimoto, Cook and Qi 27 ). The expression level of PPAR-α in the liver was increased in the HFD+FK group compared with the HFD group (Fig. 4). Furthermore, compared with the HFD group, the administration of FK-23 under the HFD condition tended to increase the expression levels of CPT-2 and trifunction, which are involved in mitochondrial fatty acid β-oxidation and are downstream target genes of PPAR-α( Reference Rakhshandehroo, Knoch and Muller 28 ). In contrast, the expression levels of ACC and FAS, which are involved in de novo lipogenesis in the liver, were up-regulated by the administration of FK-23 under the HFD condition compared with the HFD group (Fig. 4). Despite the up-regulation of these genes, the accumulation of lipid droplets was significantly decreased in the HFD+FK group compared with the HFD group (Fig. 1). This might be explained by the decreased level of blood glucose in the HFD+FK group compared with the HFD group (Table 3), since excess glucose is generally utilised for de novo lipogenesis. PPAR-γ, a member of the superfamily of nuclear receptor transcription factors, plays a major role in the metabolism of lipid and glucose, and the activation of PPAR-γ by synthetic ligands such as pioglitazone leads to a reduction in the level of blood sugar( Reference Kemnitz, Elson and Roecker 29 ). The increased expression level of PPAR-γ in the liver by the administration of FK-23 under the HFD condition could be correlated with the reduction in blood glucose level (Fig. 4 and Table 3). Our data suggest that FK-23 protects against HFD-induced hepatic steatosis by the prevention of lipid droplet accumulation through the modulation of not only the blood glucose level, but also the expression levels of genes involved in hepatic fatty acid oxidation.
In the present study, the expression levels of pro-inflammatory genes (TNF-α and MCP) were increased after feeding the HFD compared with the SD group, but FK-23 supplementation did not affect the levels of these genes. Because hepatic inflammation and protein levels of inflammatory cytokines were not evaluated histologically in the present study, further investigations will be needed to clarify the effects of FK-23 on biological responses, especially inflammation and fibrosis, in the progression of hepatic steatosis.
Recently, it was pointed out that changes in the gut microbiota are associated with obesity and exacerbation of hepatic steatosis( Reference Yoshimoto, Loo and Atarashi 30 ). Growing evidence suggests that the alteration in the microbiota contributes to the onset of low-grade inflammation in the liver by the exposure of the microbe-derived endotoxin through the enterohepatic circulation, and inflammation is considered as one of the mechanisms of the increase in fat deposition and body weight( Reference Turnbaugh and Gordon 31 ). It has been reported that a HFD causes the dysregulation of the intestinal microbiota( Reference Turnbaugh, Hamady and Yatsunenko 32 ). In fact, oral administration of FK-23 has the ability to modulate the gut microbiota (T Shimada, Q Zhang, A Hayashi, K Fukada, Y Koga and L Cheng, unpublished results). Therefore, FK-23 could prevent or restore the HFD-induced dysregulation of the microflora, which might result in the attenuation of hepatic steatosis and the prevention of body-weight gain. Further studies on the gut microbiota are required to investigate this hypothesis.
Taken together, the present results demonstrated that oral administration of FK-23 attenuates HFD-induced hyperglycaemia and the level of alanine aminotransferase in serum, and inhibits body-weight gain and liver weight without the modulation of intestinal fat absorption. These results were consistent with the changes in the expression levels of genes involved in lipid metabolism induced by the administration of FK-23 under the HFD condition. However, the detailed mechanism of action remains unclear, and further studies are required for the understanding of the anti-obesity and anti-steatosis effects of FK-23.
Acknowledgements
The present study was supported by Grants-in-Aid for Scientific Research (C) to Y. N. (no. 25460958) from the Japan Society for the Promotion of Science, and by an Adaptable and Seamless Technology Transfer Program through target-driven R&D to Y. N. from the Japan Science and Technology Agency.
The authors’ contributions are as follows: M. K., T. S., M. M., K. K., Y. H. and M. O. carried out the animal experiments; K. M. performed the gene expression analysis; Y. N. and T. Y. advised on the interpretation of the data analysis. T. S., K. F., M. M., K. K., Y. H. and Y. N. revised the manuscript. All authors contributed to the design of the study, and read and approved the final manuscript.
Conflict of interest: Y. N. received scholarship funds from Otsuka Pharmaceutical Company Limited, Takeda Pharmaceutical Company Limited, Eisai Company Limited and Tanabe-Mitsubishi Company Limited. T. Y. is affiliated to a donation-funded department from AstraZeneca Company Limited, Eisai Company Limited, Otsuka Pharmaceutical Company Limited, MSD K.K., Dainippon Sumitomo Pharma Company Limited, Chugai Pharmaceutical Company Limited, FUJIFILM Medical Company Limited and Merck Serono Company Limited. T. S., M. M., and T. Y. are affiliated to the Collaborative Research Department (Department of Gastrointestinal Immunology), which receives donations from Nichinichi Pharmaceutical Company Limited. The other authors have no conflict of interest to declare.