Introduction
Deep ice drilling at both poles reveals valuable climate records extending up to 800 ka into the past (EPICA Community Members, 2004), in addition to unveiling much about the physical structure of the Greenland, West and East Antarctic ice sheets. In recent years, ice-core drilling, transport, sampling and analytical procedures have continually improved, spurred by the goal of providing continuous, high-resolution records of atmospheric greenhouse gases and climate and environmental proxies.
However, poor ice-core quality in the so-called brittle ice zone (BIZ), where extensive fracturing of core samples is caused by rapid relaxation (decompression) of the ice (e.g. Reference GowGow, 1971), remains a technical challenge at all phases of intermediate-depth and deep ice-core studies. At the drill site, mechanical stresses from drilling and logging the core can degrade initial core quality in this zone. Sampling at freezer facilities often induces further fracturing as individual brittle ice-core samples are cut with bandsaws. Finally, brittle ice samples prove challenging for laboratory procedures such as continuous-flow analysis (e.g. Reference Osterberg, Handley, Sneed, Mayewski and KreutzOsterberg and others, 2006; Reference Bigler, Svensson, Kettner, Vallelonga, Nielsen and SteffensenBigler and others, 2011), with fractures allowing drilling fluid required for deep ice drilling to penetrate into samples, contaminating major-ion chemistry, trace chemistry and gas measurements.
While at relatively high-snow-accumulation deep drill sites ice ages in the BIZ represent several thousand years of the Holocene, high-snow-accumulation intermediate-depth drill sites and low-snow-accumulation deep drill sites may place many thousands of years of transitional and/or glacial ice within this zone of compromised core quality. In the case of drill sites with intermediate ice thickness, a large fraction (90% or more) of the dated ice-core record will likely be placed within the BIZ. Brittle ice-core quality, through recovery, transport, sampling and analysis, is one of the technical challenges identified by the International Partnerships in Ice Core Sciences (IPICS), with implications both for the ‘40 k array’ initiative to gather a spatially distributed, bipolar network of 40 ka ice-core records, and any Antarctic ‘oldest ice’ site chosen to retrieve a continuous 1.5 Ma record (IPICS, 2005; Reference Brook, Wolff, Dahl-Jensen, Fischer and SteigBrook and others, 2006).
The term ‘brittle ice’ with respect to ice drilling originates in the description by Reference Gow, Ueda and GarfieldGow and others (1968) of highly fractured ice from depths between 400 and 900 m in the Byrd Station ice core, the first drilled to bedrock in Antarctica. Investigation of ice relaxation by Reference GowGow (1968, Reference Gow1971), also in the Byrd Station ice core, indicates that the linear increase in overburden pressure with depth accordingly increases the in situ pressure within air bubbles in the ice; once removed from this high-pressure environment, bubble pressure exceeds the tensile strength of the confining ice, causing cracking between bubbles and initiating widespread fracturing in ice cores (Reference Uchida, Duval, Lipenkov, Hondoh, Mae and ShojiUchida and others, 1994; IPICS, 2005). Immediately upon exposure at the surface, fracturing begins explosively, propagating across- and along-core on decimeter scales. Slower relaxation of ice cores over periods of months to years after drilling (also explored by Reference GowGow, 1971) may alleviate some or all remaining brittleness; however, ice cores from Siple Dome, Antarctica, remain brittle more than a decade after cores were retrieved (personal communication from M. Twickler, 2013). Brittle fracture in ice cores only fully diminishes at depths where air bubbles are absorbed into the ice, transitioning to air-hydrate crystals (clathrates). Here the ice is more ductile as dissociation pressure and temperature is reached, incorporating gases into the crystal lattice (e.g. Reference MillerMiller, 1969; Reference Shoji and LangwayShoji and Langway, 1982; Reference Pauer, Kipfstuhl and KuhsPauer and others, 1995; Reference Kuhs, Klapproth, Chazallon and HondohKuhs and others, 2000; Reference Lipenkov and HondohLipenkov, 2000). This transition occurs gradually as bubble number-densities decrease and clathrates become dominant (e.g. Reference Uchida, Duval, Lipenkov, Hondoh, Mae and ShojiUchida and others, 1994; Reference Kipfstuhl, Pauer, Kuhs and ShojiKipfstuhl and others, 2001; Reference UeltzhöfferUeltzhöffer and others, 2010). It is expected that clathrate formation, and thus onset of ductile
The BIZ, though observed at all deep drilling sites, has seldom been specifically examined and is only approximately defined as a zone beginning several hundred meters below the ice surface and extending to depths between 1000 and 1500 m. This is in part due to the inherently qualitative definition of brittle ice, wide-ranging metrics for ice-core quality, rounding of reported depths, and the entangled effects of ice physical properties and stresses (mechanical and thermal) induced during drilling, on-site handling and transport. BIZ depths collated here are likely accurate to ±50 m. The first sustained decline in ice-core quality should be reported, and is considered here, as the top depth of the BIZ, with the bottom depth marked by a return to consistently excellent ice-core quality (e.g. fig. 10 of Reference SouneySouney and others, 2014). This paper compiles reported information regarding the BIZ for 18 deep (>1500 m) and intermediate-depth (500–1500 m) polar ice-drilling projects (Fig. 1a and b). Two effects are explored with possible controls on brittle ice onset and relief. First, the effect of varying firn-column thickness on overburden pressure at depth is considered using modeled firn-column densities at all sites. Second, reported brittle ice depths are examined with respect to clathrate stability using in situ ice temperature from borehole measurements and modeled overburden pressures. Techniques are detailed that have been or could be employed to reduce brittle fracture in ice cores during drilling, transport and sampling, and challenges pertaining to analysis of brittle ice samples are also discussed.
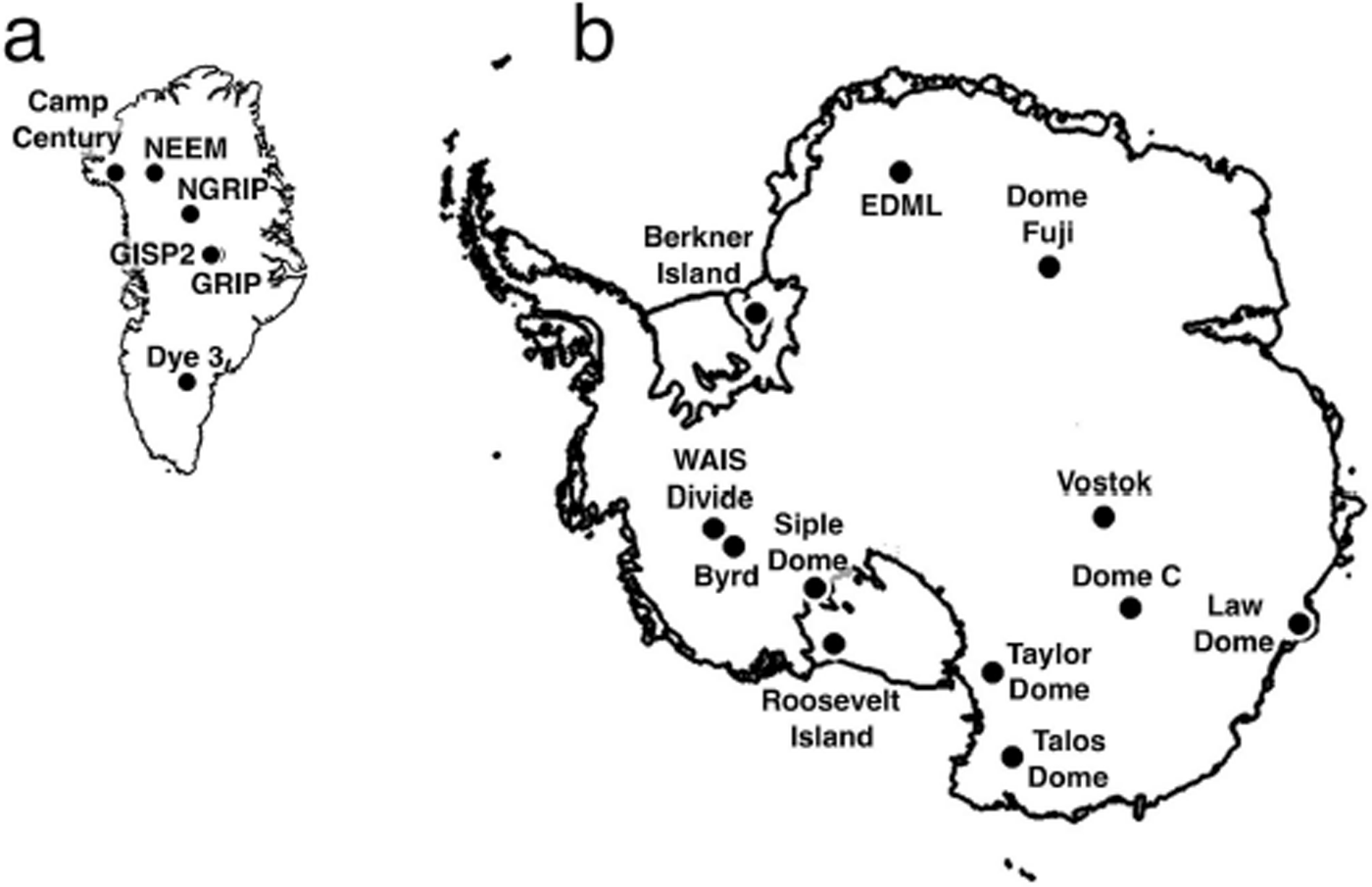
Fig. 1. Locations of polar drill sites in (a) Greenland and (b) Antarctica discussed in text. Map data from Reference TimmermannTimmermann and others (2010). ice and improved ice-core quality, occurs at shallower depths as ice temperature decreases (Reference MillerMiller, 1969).
Brittle Ice Zone Depth
Reported BIZ depths and drill site conditions – snow accumulation rate, mean annual surface air temperature and firn/ice transition (FIT) depth – are summarized in Tables 1, 2 and 3 for sites across the Greenland ice sheet (GIS), West Antarctic ice sheet (WAIS) and East Antarctic ice sheet (EAIS), respectively. BIZ top and bottom depths, as well as FIT depth (assuming a density of 830 kg m−3), are displayed for all sites in Figure 2. While intermediate-depth drilling projects at Siple Dome, Berkner Island, Roosevelt Island, Law Dome and Taylor Dome experienced brittle ice conditions, the ice/bed interface was encountered before reaching pressures and temperatures suitable for clathrate formation and the transition to ductile ice. Some ice-core quality improvement was anecdotally observed in the final several meters of the Siple Dome, Berkner Island and Roosevelt Island ice cores, possibly due to higher temperatures near the bed (Reference Gow and MeeseGow and Meese, 2007; Reference Mulvaney, Alemany and PossentiMulvaney and others, 2007; personal communication from N. Bertler, 2013).

Fig. 2. Firn/ice transition (FIT; depth where density 830 kg m−3 reached; circles), BIZ top depths (inverted triangles) and BIZ bottom depths (triangles) at ice-drilling sites, ordered by increasing FIT depth. Open triangles denote BIZ bottom depths which represent the ice/bed interface, rather than transition to the bubble-free, ductile ice zone. RICE: Roosevelt Island Climate Evolution; WSD: WAIS Divide; GISP2: Greenland Ice Sheet Project 2; GRIP: Greenland Ice Core Project; NGRIP: North Greenland Ice Core Project; NEEM: North Greenland Eemian Ice Drilling; EDML: EPICA Dronning Maud Land; EDC: EPICA Dome C.
Table 1. Site information, brittle ice zone (BIZ) depths (bold), mean annual snow accumulation, mean surface air temperature, firn/ice transition depth (density 830 kg m−3 reached) and BIZ ice ages for Greenland ice sheet ice-drilling sites. GRIP: Greenland Ice Core Project; GISP2: Greenland Ice Sheet Project 2; NGRIP: North Greenland Ice Core Project; NEEM: North Greenland Eemian Ice Drilling
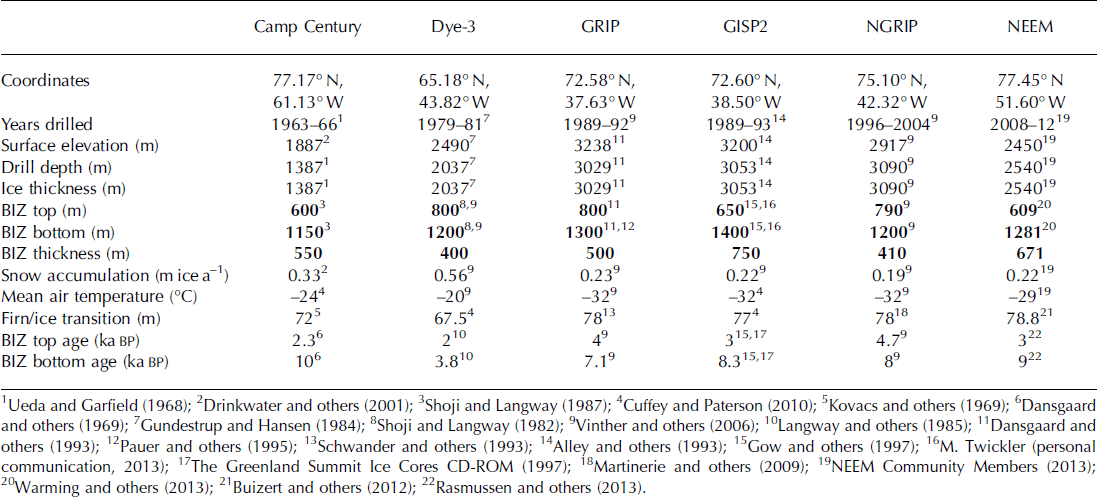
Table 2. Site information, BIZ depths (bold), mean annual snow accumulation, mean surface air temperature, FIT depth (density 830 kg m−3 reached) and BIZ ice ages for WAIS ice-drilling sites

Table 3. Site information, BIZ depths (bold), mean annual snow accumulation, mean surface air temperature, FIT depth (density 830 kg m−3 reached) and BIZ ice ages for EAIS ice-drilling sites

Pressure and temperature effects: brittle zone onset
Snow accumulation rate and surface air temperature are primary controls on the densification of polar firn (e.g. Reference Herron and LangwayHerron and Langway, 1980; Reference Ligtenberg, Helsen and Van den BroekeLigtenberg and others, 2011), although the firnification process is not fully understood (Reference Hörhold, Kipfstuhl, Wilhelms, Freitag and FrenzelHörhold and others, 2011). Firn densification is relevant here for its role in isolating air bubbles from porous firn and because the thickness and density of the firn column controls the exact overburden pressure imposed on ice and air bubbles at depth. Overburden pressure may affect where brittle fracture of ice cores commences, as thin firn columns at warmer, higher-snow-accumulation sites should reach full ice density (920 kg m−3) more rapidly and thus exert more overburden pressure on ice and air bubbles at depth, and vice versa. Additionally, overburden pressure and in situ ice temperature control clathrate stability and are expected to affect the depth of the transition out of the bubbly BIZ and into the bubble-free, ductile clathrate-ice zone below, with clathrates stabilizing at lower (higher) pressures when temperatures are low (high) (e.g. Reference MillerMiller, 1969).
To quantify the influence of firn column thickness on overburden pressure at depth, while also converting BIZ depths to equivalent overburden pressures, firn-column density is modeled using the University of Washington Firn Model Intercomparison Experiment online Reference Herron and LangwayHerron and Langway (1980) model (FirnMICE, http://firny.ess.washington.edu/communityfirnmodel/). A surface snow density of 390 kg m−3 is assumed for all sites, and mean annual surface air temperature and snow accumulation rate (ice equivalent) are input into the model to construct 1 m resolution depth-density profiles from the surface to 300 m for each site (Fig. 3a). Constant ice density of 920 kg m−3 is assumed below 300 m, although for bubbly ice this may be an overestimate of several kgm−3. Snow density measurements from Taylor Dome, Berkner Island and WAIS Divide agree well with model data generated for these sites (density measurements provided with the FirnMICE online model, not shown). Overburden pressure at a given depth is calculated as the sum of overlying snow and ice with density prescribed by the FirnMICE model output. One cubic meter of ice (density 920 kg m−3) exerts 9.02 × 10−3 MPa overburden pressure (Fig. 3b). Modeled overburden pressure at 300 m, the depth at which the thickest modeled firn column (Dome Fuji) reaches ice density of 920 kg m−3, is presented in Figure 3c for all sites, plotted against modeled and observed FIT depths (830 kg m−3 density). The mean misfit between observed and modeled FIT depth is −4%, with minimum misfits of +1% and −2% (EPICA Dome C and WAIS Divide, respectively) and a maximum misfit of−25% (Siple Dome).

Fig. 3. Results of FirnMICE firn column density modeling (Reference Herron and LangwayHerron and Langway (1980) model) and overburden pressure calculations. (a) Density–depth profiles generated for the 18 drill-site temperature and snow accumulation regimes, shaded according to modeled FIT depth (light grey: model FIT <70 m; grey: 70 m < model FIT < 80 m; black: model FIT >80 m); bold dashed line indicates ice density (920 kg m−3). (b) Ice overburden pressure versus depth in the firn column (0–300 m) for the 18 drill sites (light grey: model FIT <70 m; grey: 70 m < model FIT < 80 m; black: model FIT >80 m); bold dashed line indicates overburden pressure assuming constant ice density from the surface. (c) 300 m depth overburden pressure versus FIT depth from modeled (open circles) and measured (filled circles) firn-column density data.
Across the 18 drill sites, 300 m overburden pressure varies from 2.36 MPa at Dome Fuji (modeled FIT depth 114 m, +12% misfit) to 2.56 MPa at Siple Dome (modeled FIT depth 46 m, −25% misfit; Fig. 3b and c). Poor FIT depth reproduction for these end-member sites (Fig. 3c) suggests an overestimate of the possible range of overburden pressures; more conservative is 2.4 MPa at EPICA Dome C (modeled FIT depth 101 m, +1% misfit) to 2.55 MPa at Berkner Island (modeled FIT depth 52 m, −10% misfit). This overburden pressure fluctuation at depth, a maximum difference of ∼0.15–0.2 MPa between the thickest firn column (slower firnification, lower overburden pressure at depth) and the thinnest (faster firnification, higher overburden pressure at depth), is equivalent to 16.5–22.2 m of ice overburden. If the firn column is ignored and full ice density is assumed from the surface, overburden pressure at depth (2.7 MPa at 300 m) is overestimated by 0.14–0.34 MPa or ∼15.5–37.7 m of ice (dashed line, Fig. 3a and b). This results in an underestimation of the depth for theoretical clathrate stability. The BIZ does generally occur at shallower depths where the firn/ice transition is shallower (e.g. Fig. 2) and exerts greater overburden pressure at depth. This is most clearly observed at GIS and WAIS drill sites, although with low significance, as is expected due to low precision of reported BIZ depths and complex temperature and accumulation effects on firnification and clathrate formation processes. Reported BIZ top depths begin ∼10 m deeper per 1 m thickening of the FIT at the 11 GIS and WAIS drill sites (regression of GIS and WAIS FIT depths and BIZ top depths gives R 2 = 0.54, not shown). As overburden pressure alone cannot explain such a deepening, this suggests that unidentified firnification processes likely exert significant control on BIZ onset depth. Proportional deepening of BIZ onset is not observed at all EAIS drill sites, especially not those on the EAIS plateau, perhaps because of increasing tensile strength of ice at smaller grain sizes and lower temperatures (e.g. Reference ButkovichButkovich, 1954; Reference PetrovichPetrovich, 2003), as well as other unknown effects associated with extreme low-temperature and low-accumulation firn densification, grain growth and air-bubble formation.
Pressure and temperature effects: brittle zone relief
In situ ice temperature determines the depth (pressure) of clathrate stability and thus should affect the depth of the transition from bubbly, brittle ice to bubble-free, ductile ice. This is supported by observations of shallower appearance of clathrates and disappearance of air bubbles in ice cores at low-temperature sites (e.g. observations from Dye-3 and GRIP versus Vostok and Dome Fuji; Reference Ikeda-Fukazawa, Hondoh, Fukumura, Fukazawa and MaeIkeda-Fukazawa and others, 2001). While temperature effects controlling BIZ onset are less clear, it is expected that the BIZ will be relieved at shallower depths (lower pressures) where ice temperatures are lower. Using overburden pressure as calculated above, and borehole temperature data from selected drill sites (Table 4), the BIZ can be evaluated with respect to temperature and pressure and thus compared more accurately with theoretical clathrate stability (Fig. 4). BIZ onset in many cases begins at pressures (depths) where clathrates should already begin to stabilize, but brittle fracture is not relieved until ductile conditions are reached as bubble number densities become sufficiently low and clathrates dominate, strengthening ice cores. While it is tempting to suggest that BIZ bottom pressure indeed decreases with lower ice temperatures, this is not a statistically significant feature including all reported BIZ data (excluding intermediate-depth sites where clathrates do not stabilize before the ice/bed interface is reached). Certainly the BIZ in the Greenland summit ice cores and at WAIS Divide (1200–1400 m BIZ bottom depths; ∼10.5–12.5 MPa) extends several hundred meters deeper than that of Dome Fuji, Vostok, EPICA Dronning Maud Land and Talos Dome (840–1050 m BIZ bottom depths, 7.2–9.2 MPa), but the EPICA Dome C ice core remained highly fractured to 1200 m depth (10.5 MPa). Additionally, the BIZ at Camp Century, Dye-3 and Byrd Station transitions to ductile ice at shallow depths, with pressures only 1–2 MPa greater than that required for initial clathrate stability, while most sites transition out of the BIZ at pressures 3–5 MPa in excess of requirements for theoretical onset of clathrate stability (Fig. 4). This raises the interesting prospect that ice-flow advection of cold ice towards the surface at flank sites could encourage shallower formation of clathrates (e.g. Camp Century, Byrd Station; Reference Shoji and LangwayShoji and Langway, 1987). However, without disentangling the effects of imprecise BIZ records, drill performance and ice-core handling/transport, it is difficult to use reported brittle ice depths to further evaluate this correlation between clathrate stability and the bottom of the BIZ.
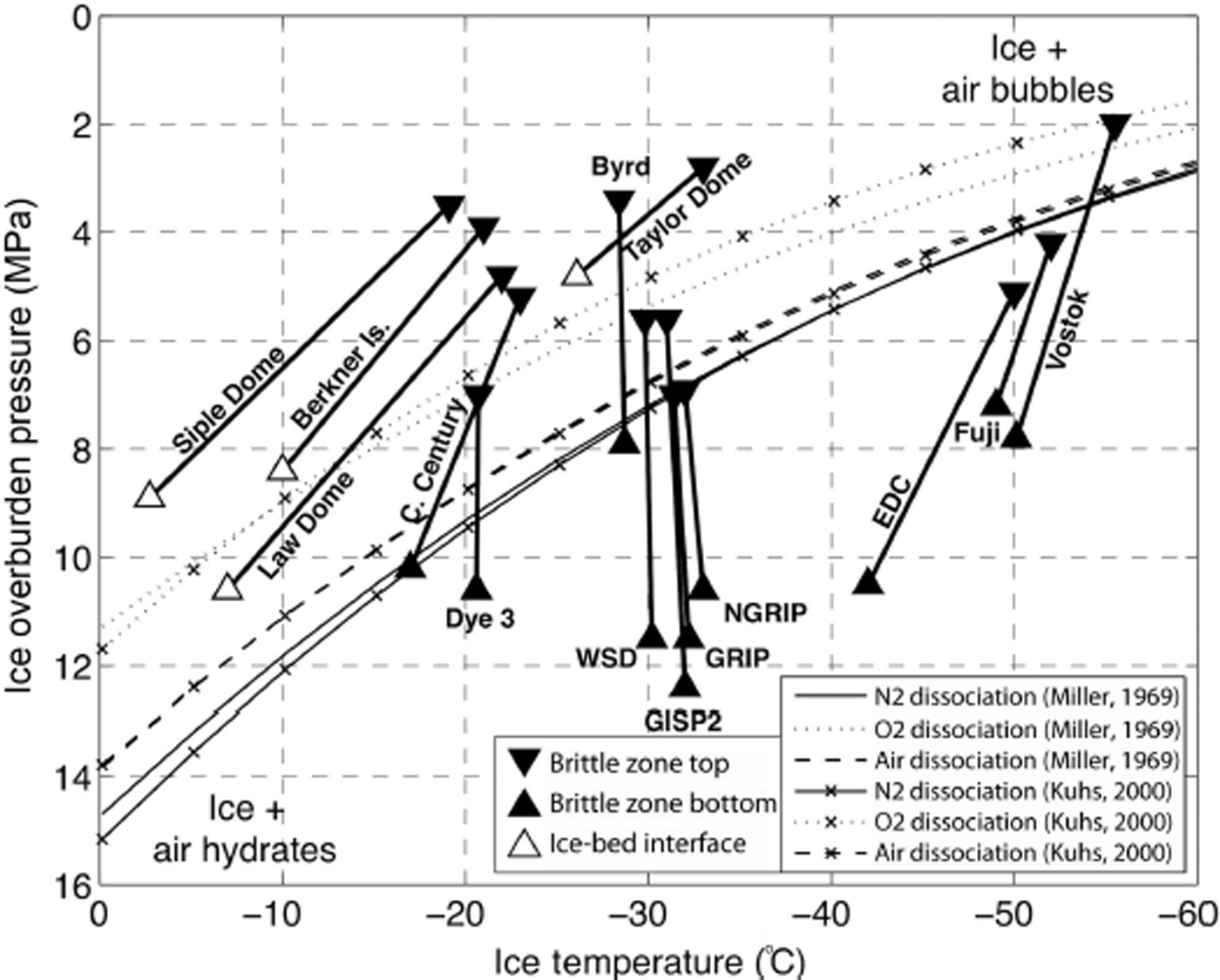
Fig. 4. BIZ top (inverted triangles) and bottom pressures (triangles) plotted at respective in situ ice temperature from borehole temperature measurements (Table 4). Theoretical clathrate stability curves are plotted for N2 (solid), O2 (dotted) and air (dashed) hydrates (Reference MillerMiller, 1969; Reference Kuhs, Klapproth, Chazallon and HondohKuhs and others, 2000). The stability curve of Reference Kuhs, Klapproth, Chazallon and HondohKuhs and others (2000) is indicated with ‘x’. Open triangles denote BIZ bottom pressures at the ice/bed interface, thus not the full transition from the bubbly, brittle-ice zone to the bubble-free, ductile ice zone below. WSD: WAIS Divide; EDC: EPICA Dome C.
Table 4. In situ ice temperature (borehole temperature; °C) at BIZ top and bottom depths (see Tables 1–3) for selected sites. Temperatures are ±0.5°C, estimated from published graphical data where original datasets could not be accessed. Temperature data for Berkner Island are modeled
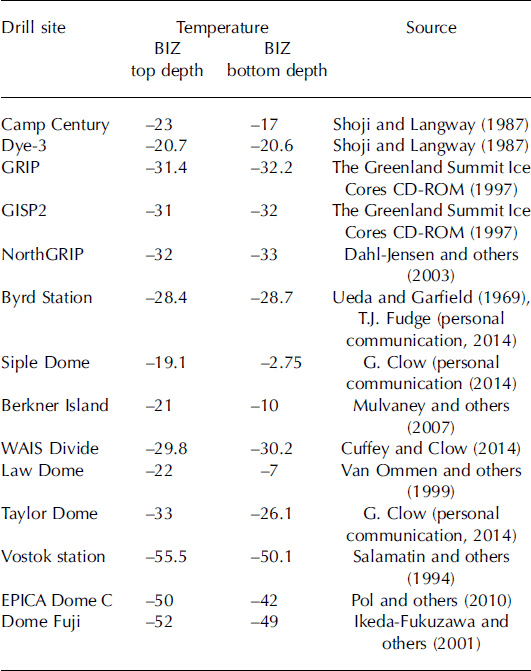
To improve understanding of the mechanisms involved in BIZ onset and relief, it may prove useful to investigate ice physical properties including bubble number density, bubble size, micro-bubbles and ice fabric (e.g. grain size, crystal anisotropy). Take the anomalously shallow BIZ at Vostok, for example: Reference Uchida, Duval, Lipenkov, Hondoh, Mae and ShojiUchida and others (1994) observed a reduction of core quality caused by fractures as shallow as 100 m (likely linked to thermal drilling at Vostok), becoming heavily fractured from 250 to 750 m with progressive improvement to 900 m where core quality again became excellent. This shallowest occurrence of the BIZ may be related to a rapid increase in bubble number density (bubbles cm−3) observed in the Vostok ice core beginning at ∼300 m depth (increasing from ∼400 to ∼800 bubbles cm-3; Reference Uchida, Duval, Lipenkov, Hondoh, Mae and ShojiUchida and others, 1994; Reference UeltzhöfferUeltzhöffer and others, 2010). Such an increase in bubble number density may effectively reduce bubble pressures required for fracture propagation by narrowing interstitial ice between bubble cavities. Bubble number densities reported in the BIZ at Byrd Station and WAIS Divide were relatively more constant at ∼200 and ∼450 bubbles cm−3, respectively (Reference GowGow, 1971; Fitzpatrick and others, in press). Micro-bubbles formed through sublimation-condensation processes may also play a role at Vostok (e.g. Reference Lipenkov and HondohLipenkov, 2000), and are observed in the EPICA Dome C and Dronning Maud Land ice cores (Reference UeltzhöfferUeltzhöffer and others, 2010). The second shallowest occurrence of the BIZ, 335 m at Taylor Dome, may be anomalously shallow due to high strain rates at this site; elongated bubbles were observed from 360 to 390 m (Reference FitzpatrickFitzpatrick, 1994).
Brittle Ice Zone Age
Figure 5 displays observed BIZ top and bottom ages from published age scales developed for the 18 drill sites discussed above (detailed ages in Tables 1–3). At deep ice-core sites where snow accumulation rates are relatively high (e.g. GIS and inland WAIS sites), the age of ice within the BIZ is relatively young, dating from as few as 2 kaBP (Dye-3; Reference Langway, Oeschger and DansgaardLangway and others, 1985) to 9.5 ka BP (Byrd Station; Reference Blunier and BrookBlunier and Brook, 2001). These ages represent ∼10% or less of the age of dated ice-core records developed from these sites. While the Holocene marks the beginning of relatively stable global climate, with the exception of the 8.2 ka BP event (e.g. Reference Alley, Mayewski, Sowers, Stuiver, Taylor and ClarkAlley and others, 1997), continued emphasis on understanding natural climate variability precludes classifying ice of this age as scientifically less interesting than older transitional and glacial ice (e.g. Reference MayewskiMayewski and others, 2004; Reference Marcott, Shakun, Clark and MixMarcott and others, 2013; Reference SteigSteig and others, 2013).

Fig. 5. BIZ top (inverted triangles) and bottom (triangles) age (kaBP) at drill sites, ordered by region and date of drilling. Open triangles denote BIZ bottom ages which represent the maximum dated age (∼ice/bed interface) at these sites. WSD: WAIS Divide; RICE: Roosevelt Island Climate Evolution; EDC: EPICA Dome C; EDML: EPICA Dronning Maud Land.
A wide range of ice ages is found within the BIZ at deep ice-core sites with low snow accumulation rates (e.g. EAIS plateau sites: Taylor Dome, Vostok, Dome C, Dronning Maud Land, Dome Fuji, Talos Dome), and intermediate-depth ice-core sites with higher snow accumulation rates (e.g. coastal Antarctic sites: Siple Dome, Berkner Island, Roosevelt Island, Law Dome). In intermediate-depth ice-core records (all at intermediate ice-thickness coastal Antarctic locations), ice at the top of the BIZ dates to between approximately 4 ka BP at Roosevelt Island (personal communication from N. Bertler, 2013) and 9 ka BP at Law Dome (Reference Morgan, Wookey, Li, Van Ommen, Skinner and FitzpatrickMorgan and others, 1997). Ice at these coastal Antarctic sites remains brittle to the bed, dating to a minimum of ∼40 ka BP (Roosevelt Island; personal communication from N. Bertler, 2013) and in all cases placing at least 90% of the dated ice-core record within the BIZ. Brittle ice at EPICA Dome C dates from 29 to 80.5 ka BP (EPICA Community Members, 2004). While not a large fraction of the entire ice-core record (∼6% of the 800 ka record), this section of the Dome C ice core is highly detailed when compared to deeper ice where ice-flow thinning impairs resolution at this extremely low-accumulation site. From the surface to ∼2000 m depth, 55 cm long sections of the Dome C ice core exhibit temporal resolution of 50–100 years or less, while below 2000 m similar sections contain 200–1000 years or more (Reference PolPol and others, 2010). Taylor Dome, a very shallow site, became brittle from a depth of 335 m, dated to 10 kaBP, remaining brittle to the bed and spanning ice ages in excess of 160 ka BP (Reference SteigSteig and others, 2000). Ice within the BIZ at these coastal Antarctic and EAIS plateau sites spans ages of fundamental interest to paleoclimate research, relevant to investigations into regional timing of the onset of deglaciation during the late-Pleistocene and rapid climate anomalies occurring during this transition (e.g. Younger Dryas, Antarctic Cold Reversal; Reference AlleyAlley and others, 1993; WAIS Divide Project Members, 2013).
Mitigating Brittle Ice Impacts
Drilling techniques
Currently, drilling through the BIZ produces ice-core sections with several to many breaks, fractures and hairline cracks, commonly affecting more than half of recovered ice in the middle of this zone. Drilling fluid required at these depths pervades all cracks and fractures, contaminating many chemical analyses. At WAIS Divide, where BIZ core quality was the best of any recent United States-led drilling project, 1 m long core sections from 900 to 1200 m were on average between ‘good’ (containing zero to three breaks or 50% unfractured) and ‘fair’ quality (containing >10 cm of core length without fractures; Reference SouneySouney and others, 2014).
As the only step performed at in situ pressures within the ice sheet (minimum of 2.0 MPa, maximum 12.4 MPa in the BIZ), with the added benefit of damping effects associated with a liquid-filled borehole, the drilling process provides important opportunities to reduce the major component of brittle fracture in ice cores by performing mechanically severe steps that might damage cores if performed at surface pressures (∼700 hPa, 0.07 MPa). For example, drilling at WAIS Divide employed a unique strategy specifically for brittle ice, performing three core breaks per ∼2.5 m drill run before returning the drill sonde to the surface (detailed in Reference SouneySouney and others, 2014). This technique of performing several core breaks per run at in situ pressure results in ice-core sections that are ready to ship, without subjecting brittle ice cores at surface pressure to the vibration and high stresses of making circular-saw section cuts. A similar downhole technical innovation suggested by Reference UedaUeda (2002) is the development of a drill sonde that captures and seals cores in a vessel at in situ pressures, potentially allowing for a more gradual transition to atmospheric pressure than the usual minutes-to-hours-long transition as the drill sonde is brought to the surface. Slowing the pressure and temperature transition by hoisting brittle ice-drill runs slowly to the surface, reducing drill penetration rate and considering drill fluid pressure balance have also been proposed (see the discussion by J. Schwander and others in IPICS, 2005).
Maintaining temperatures within drilling and on-site storage structures similar to those at depth in the borehole is a commonly practised technique in ice drilling (e.g. Reference SouneySouney and others, 2014). At Roosevelt Island, an actively cooled storage cave kept ice at −23°C despite surface temperatures reaching as much as −5°C (personal communication from D. Mandeno, 2014). Temperature gradients may reach several tens of degrees between in situ ice temperature and surface drill structure temperatures, which will cause differential heating of ice cores brought to the surface, inducing temperature and stress gradients as the ice-core surface warms (and expands) more quickly than the interior. However, it is difficult to overstate the primary impact of the pressure gradient between the BIZ and the surface, which, as a ratio, is a minimum of 30 : 1 at the shallowest observed BIZ onset (Vostok: 2.0 MPa at 250 m, ∼0.07 MPa surface air pressure) and grows to more than a 100 : 1 ratio at most ice-core drill sites (reaching a maximum BIZ bottom-to-surface-pressure ratio of 177 : 1 at the bottom of the Greenland Ice Sheet Project 2 (GISP2) BIZ: 1400 m depth, 12.4 MPa).
Transport techniques
Reduction of mechanical shock during core transport from the drill site to laboratories has been achieved primarily by sheathing brittle ice cores in tight-fitting nylon netting immediately upon removal from the drill, and delaying shipment of brittle ice for as long as logistically possible to allow for maximum relaxation. Use of netting was innovated at Berkner Island (Reference Mulvaney, Alemany and PossentiMulvaney and others, 2007), and the same was used successfully at WAIS Divide (Reference SouneySouney and others, 2014) and Roosevelt Island (personal communication from N. Bertler, 2013). While itself not preventing initial fracture of brittle ice cores, nylon netting holds badly fragmented sections in place, preventing any loss of stratigraphic order, and protects cores from further damage due to vibration during shipment.
Relaxation of ice cores after drilling is examined thoroughly by Reference GowGow (1971), measuring significant post-drilling volume expansion (density reduction) at all depths in the Byrd Station ice core. Cores from the BIZ around 800 m depth exhibit greatest expansion: ∼0.2% (0.002 kg m−3 density decrease) after 8 months, ∼0.4% (0.004 kg m−3 decrease) after 16 months, and up to ∼0.6% (0.006 kg m−3 decrease) after 27 months (see fig. 2 of Reference GowGow, 1971). Cores from other depths expanded by an average of ∼0.2% (0.002 kg m−3 decrease) after 27 months. Nearly identical relaxation characteristics were observed in the GISP2 ice core (Reference GowGow and others, 1997). Ice cores retrieved using a hot-water drill at Siple Dome relaxed very quickly, likely due to the thermal drilling technique, but cores were also stored at relatively high surface temperatures after drilling (Reference Engelhardt, Kamb and BolseyEngelhardt and others, 2000; Reference Gow and MeeseGow and Meese, 2007). Ice cores retrieved by PICO (Polar Ice Coring Office) mechanical drilling atSiple Dome showed very little relaxation, and remain brittle to date (Reference Gow and MeeseGow and Meese, 2007; personal communication from M. Twickler, 2013).
Much of this relaxation in brittle ice is attributed to slow dilation of highly pressurized bubbles abundant in this zone. This lends support to the practice of overwintering brittle ice, most recently performed at WAIS Divide (Reference SouneySouney and others, 2014). At Roosevelt Island, ∼2 m long drill runs were sheathed in netting and stored in a refrigerated snow cave for up to 14 days before making 1 m section cuts for shipment. Improvements were noted in bandsaw cuts after even this short period of relaxation, although some propensity for fracturing remained (personal communication from N. Bertler, 2013). It is important to note that overwintering or long-term storage of brittle ice cores delays sampling and analysis of this section of ice. While this delay is less significant on the time frame of a multi-year deep drilling project, for smaller endeavors, especially at intermediate-depth coastal sites where a significant portion of the dated ice-core record lies within the BIZ, delaying shipment and/or sampling of ice cores is more challenging.
Sampling techniques
Ice-core sampling is commonly performed at −20 to −25°C, making preliminary longitudinal cuts using a horizontal bandsaw and subsequent sampling with common vertical bandsaws. While a simple tool, the bandsaw applies consistent force at the cutting teeth, especially if tracking of the saw along-core is automated to steadily move the saw blade through the ice. After sufficient relaxation – allowing for slow dilation of air bubbles long after initial violent cracking and fracturing observed immediately after drilling–brittle ice may feed through a bandsaw with little additional fracturing. Brittle ice below depths of 475 m from Roosevelt Island proved prohibitively brittle after 6 months stored at −30°C, with several instances of near-catastrophic fracturing during horizontal cutting due to vibration from the saw blade. At this depth, a conventional vertical bandsaw used to make ∼0.035 m × 0.035 m × 1.0 m rods of ice also began to add many fractures to previously flawless ice-core samples, which had immediately prior been cut into ∼0.1 m × 0.035 m × 1.0 m slabs without damage. Core sampling was halted at 500 m and the remaining ice stored at −18°C for an additional year, after which cutting proceeded without significant challenges.
Other cutting instruments may prove more conducive to processing brittle ice-core samples. Reference TisonTison (1994) describes the use of a diamond-wire saw for preparing thin sections of debris-rich or brittle ice, an option attractive for its reduced vibration levels. However, with slow cutting rates and the high cost of diamond wires, this option may require significant development before being applicable to high-volume ice-core sample processing.
Analytical techniques
Many analyses performed in polar ice-core studies depend on relatively unbroken samples to prevent contaminants from altering original paleoclimate or paleo-environmental signals. Measurements of atmospheric gases preserved in bubbles ideally require avoidance of section cuts and other breaks present in ice-core samples, in order to exclude modern atmospheric gases from analysis. Major-ion and trace chemistry, analyzed in longitudinal samples from the center of ice cores in order to avoid modern contaminants imparted during drilling, shipment and sampling, requires removal of outer sample surfaces and cleaning of any exposed surfaces including section cuts and fractures. Drilling fluid pervades all fractures in ice-core samples – especially fluids with low volatility, such as Estisol-240 (Dow Haltermann, Germany) coconut oil extract used at NEEM (North Greenland Eemian Ice Drilling) and Roosevelt Island – rendering chemical analyses extremely difficult, especially in ice from the BIZ.
Continuous-flow analysis (CFA) systems gravity-feed longitudinal ice samples through a sectioned heating plate, pumping meltwater directly into online instruments and/or fraction collectors to create discrete sub-samples (e.g. Reference Osterberg, Handley, Sneed, Mayewski and KreutzOsterberg and others, 2006; Reference Bigler, Svensson, Kettner, Vallelonga, Nielsen and SteffensenBigler and others, 2011). When analyzing highly fractured samples in CFA systems, contamination affects not only fractured core sections, but also subsequent ice as relatively high-concentration contaminants wash out of sample lines and instruments. Additionally, vertical guide systems for gravity-feeding samples struggle to accommodate ice samples containing fractures, especially high-angle longitudinal fractures, which commonly wedge against plastic guides, disrupting sample flow and accurate depth logging.
Fractured sample sections are commonly removed entirely from CFA campaigns, such that CFA for major-ion chemistry of brittle ice from the WAIS Divide ice core only processed ∼62% of ice from the depths of 577 m to 1300 m (personal communication from D. Ferris, 2014). An optical drill fluid detection system identified 27 instances of drill fluid contamination in CFA tubing while analyzing 175 m of brittle ice from the NEEM ice core (Reference Warming, Svensson, Vallelonga and BiglerWarming and others, 2013). Using this detection system, particular negative impacts were noted for dust, conductivity, ammonium, hydrogen peroxide and sulfate datasets in brittle ice from the NEEM ice core. While technically challenging, feasible solutions have been developed for analyzing brittle ice-core samples with little sample loss, such as the high-resolution CFA water stable-isotope analysis of 13 mm × 13 mm × 1.0 m rods of ice from the WAIS Divide ice core (B. Vaughn and others, unpublished information). This approach used tightly fitting square acrylic tubes to protect the fragile ice rods during shipment, and also support them vertically in a sample rack during analysis – while light vibration successfully prevented wedging against the acrylic tubing during melting, even in highly fractured brittle ice (personal communication from B. Vaughn, 2014).
Conclusion
At 18 intermediate-depth and deep polar ice-core drilling sites across the Greenland ice sheet and West and East Antarctic ice sheets, the BIZ of poor-quality, highly fractured ice cores extends from a mean top depth of 545 ± 162 m to a mean bottom depth of 1132 ± 178 m (excluding intermediate-depth sites where the ice/bed interface is reached before the transition to ductile ice).
Firn-column thickness, controlled primarily by site temperature and snow accumulation rate, determines the precise overburden pressure at depth, quantified here to demonstrate that thicker (thinner) firn columns apply less (more) pressure at depth. Both reported BIZ top and bottom depths at GIS and WAIS sites are in fact deeper where FIT depth is similarly deep, as could be expected due to fluctuating overburden pressure; however, the deepening of the BIZ is greater than can be explained from overburden pressure alone. Additionally, the absence of this relationship at extremely cold, dry EAIS plateau sites suggests that other factors associated with firn densification and grain growth, affecting eventual clathrate formation and stability, are likely involved. While it is expected, due to pressure and temperature controls on clathrate stability, that the BIZ should transition to ductile, bubble-free ice at lower pressures (shallower depths) when ice temperatures are lower, this is not a quantitative feature of reported BIZ bottom depths including all deep drill sites. Although shallower stability of clathrates and shallower disappearance of air bubbles is observed in ice cores from colder sites, BIZ bottom depths do not clearly behave similarly. This is likely due to imprecision in reported BIZ depths, as well as the convolution of ice-core fracture caused purely by physical properties during relaxation and fracture due to additional stresses induced during retrieval (e.g. drill performance, handling techniques).
Consideration of this 531 ± 138 m thick zone (mean BIZ top minus bottom depth excluding intermediate-depth sites), where pressurized air bubbles and ice relaxation upon retrieval of cores cause extensive and sometimes explosive fracturing, is pertinent to all projects developing records from ice cores recovered at depths greater than ∼400 m (i.e. mean BIZ top depth, 545 m, less one standard deviation, 162 m, gives 383 m). Relatively high snow accumulation rates and thick ice ensure that ice from the BIZ at inland GIS and WAIS sites is restricted to Holocene ages, and spans <10% of the completed ice-core records. EAIS plateau and coastal Antarctic drill sites, however, place a considerably larger amount of dated ice-core records in the BIZ. Low snow accumulation rates and large ice thicknesses at EAIS plateau sites place 20 000–50 000 years of glacial ice from these ice-core records in the BIZ, affecting the resolution of these valuable records which are already challenged by low snow accumulation rates. Conversely, high snow accumulation rates and limited ice thickness at coastal Antarctic drill sites place the majority of recovered ice and dated ice-core records within the BIZ, at least 90% of the records at all coastal Antarctic drill sites.
Innovative drilling and transport strategies have had recent successes in minimizing fracturing in ice cores from the BIZ, including the WAIS Divide brittle-ice drilling technique, netting core after removing from the drill, and relaxing ice prior to shipment and/or sampling. However, comparatively little has been done to develop better sampling methods and techniques for analyzing highly fractured ice-core samples. Consideration of mitigating strategies at any stage can have beneficial impacts on downstream work phases, most importantly potentially improving final scientific results. Targeting of ice-core sites is increasingly focused by refined scientific questions, specific research interests and desire to infill areas of sparse geographical coverage. Advancing understanding of the physical mechanisms controlling the BIZ has the potential to significantly improve the continuous recovery and development of ice-core paleoclimate and environmental records, as this zone affects samples of ages spanning periods of fundamental scientific interest at many potential drill sites.
Acknowledgements
The author thanks R. Dadic and N. Bertler for thoughtful discussion of the manuscript. Thorough critique, discussion and encouragement from two anonymous reviewers significantly improved on the original text. The online University of Washington FirnMICE model (http://firny.ess.washington.edu/communityfirnmodel/) was used to model firn density and estimate overburden pressures. The author has been funded by the New Zealand Ministry of Business, Innovation, and Employment Grants (RDF-VUW-1103, CO5X0202), Victoria University and GNS Science. This work is a contribution to the Roosevelt Island Climate Evolution (RICE) Programme, funded by national contributions from New Zealand, Australia, Denmark, Germany, Italy, the People’s Republic of China, Sweden, United Kingdom and the United States of America. The main logistic support for RICE was provided by Antarctica New Zealand (K049) and the US Antarctic Program (I-209M).