1.0 INTRODUCTION
There is a growing interest in decreasing the net emissions produced by gas turbines. This has led to research and development towards operating gas turbines using alternative fuels. The driving factors for this increased interest towards alternative fuels and decreasing emissions comes from rising fuel cost(1), decreasing crude oil resources and increasing environmental consideration(Reference Žarnovský, Petková, Drlička and Dobránsky2–Reference Rogers, Lee, Raper, Foster, Wilson and Newton4). There is also a growing concern about local air quality around airports, and steps are being taken by industry to reduce emissions. The aviation industry is growing at a rate of approximately 4% each year and is predicted to rise at this rate for decades to come(1). It is estimated that aviation currently accounts for about 3% of total global warming gases produced and is therefore continuously rising due to growth in the aviation industry. Alternative fuels derived by the Fischer-Tropsch (F-T) process from biomass, coal and gas draw an interest by researchers and industry due to their drop-in capability i.e. no engine modification required(Reference Blakey, Rye and Wilson5). Another reason for this interest is to improve the security of energy supply as alternative fuel sources can be obtained domestically.
While oil shale and F-T fuels offer increased energy security, bio-derived fuels are renewable and claimed to reduce carbon emissions in their life cycle(Reference Murugesan, Umarani, Subramanian and Nedunchezhian6). F-T fuels can be derived from a wide range of feedstocks such as natural gas, used cooking oil (UCO), fuel crops, coal, etc. F-T fuels have the potential to provide better combustion properties and lower emissions. Fuel derived from oil shale, gas and coal are non-renewable sources of fuels, but are readily available and viable as these are obtained using existing technologies. Commercial production of fuel from shale, gas and coal has been accomplished in several countries to date. In 1999, Sasol produced a semi-synthetic jet fuel (SSJF), which was a blend of up to 50% of synthetic fuel made from coal by F-T synthesis and conventional jet fuel. From that time onwards SSJF has been supplied to the Johannesburg Airport, South Africa, for use for gas turbines(Reference Hemighaus, Boval, Bosley, Organ, Lind, Brouette, Thompson, Lynch and Jones7).
According to present regulations, restrictions placed on commercial flights on the use of alternative fuels are stringent. This is due to economic and logistic issues and partly to provide safe and reliable fuel that can perform in the arduous conditions in which gas turbines work(Reference Yildirim and Abanteriba8). Since the aviation industry tends to keep its assets for around 40 years(Reference Parker and Lathoud9). The ability of alternative fuels to be used as a drop-in fuel in legacy aircraft is very important along with their ability to meet future fuel standards. The present aviation fuel specifications are laid out in the American Society for Testing and Materials (ASTM) D 1655(10). Any new fuel has to meet the specifications and has to go through a rigorous approval process as shown in Fig. 1(11). Any new alternate fuel development program must consider all other aspects of airframe, fuel handling and fuel processing as well(Reference Blazowski12).

Figure 1. Flowchart of the approval process for new aviation turbine fuels(11).
In recent years, several commercial aircrafts have performed flight demonstrations burning various blends of novel alternative fuels and conventional jet fuels. The ASTM has approved blends of up to 50% synthetic blend stocks to be used with conventional jet fuel(13). Researchers have done various studies on the use of alternative fuels in gas turbines, both for military and commercial aviation (Reference Corporan, Dewitt, Belovich, Pawlik, Lynch, Gord and Meyer14–Reference Moore, Shook, Beyersdorf, Corr, Herndon, Knighton, Miake-Lye, Thornhill, Winstead, Yu, Ziemba and Anderson26). It has also been observed in various studies that there is an advantage in using alternative blends for gas turbines as the use of paraffinic alternative fuels aids in reducing the net carbon footprint, particulate and smoke emissions(Reference Rye and Wilson27–Reference Khandelwal, Wijesinghe, Sriraman, Runchal, Gupta, Kushari, De and Aggarwal32). This can also contribute to improving the local air quality(Reference Corporan, Dewitt, Belovich, Pawlik, Lynch, Gord and Meyer14,Reference Anderson, Beyersdorf, Hudgins, Plant, Thornhill, Winstead, Ziemba, Whitefield, Hagen, Lobo, Howard, Knighton, Bulzan, Tacina, Wey, Corporan, Vander WAL, Miake-LYE, Herndon, Bhargava, Dodds, Kinsey, Lee, Santori and Liscinsky15,Reference Lobo, Hagen and Whitefield19–Reference Lee, Santoni, Wood, Herndon, Miake-Lye, Zahniser, Wofsy and Munger25) . Rye and Wilson(Reference Rye and Wilson27) studied the effect of alternative fuel composition on gas turbine ignition performance. Lobo et al. (Reference Lobo, Christie, Khandelwal, Blakey and Raper28) studied the effect of fuels derived from alternative sources on non-volatile particulate matter (PM) emissions. In recent years, studies have presented results on vibrational and noise production while using alternative fuels(Reference Khandelwal, Roy, Lord and Blakey29,Reference Khandelwal, Roy and Lord30,Reference Wijesinghe and Khandelwal33) .
There are various studies in the literature regarding the overall environmental impact, global warming potential and the use of alternative fuels in gas turbines (Reference Brem, Durdina, Siegerist, Beyerle, Bruderer, Rindlisbacher, Rocci-DENIS, Andac, Zelina, Penanhoat and Wang26,Reference Lobo, Christie, Khandelwal, Blakey and Raper28–Reference Ruslan, Ahmed and Khandelwal31,Reference Blakey, Wilson, Farmery and Midgley34–Reference Christie, Lobo, Lee and Raper47) . Recent studies by Christie et al.(Reference Christie, Lobo, Lee and Raper47) and Brem et al.(Reference Brem, Durdina, Siegerist, Beyerle, Bruderer, Rindlisbacher, Rocci-DENIS, Andac, Zelina, Penanhoat and Wang26) show the impact of hydrogen content in the fuel on PM emissions. Results of experimental tests related to the impact of gas turbine engines on the environment are not evident in the literature and not fully explored. One of the reasons for fewer studies and tests on the use of alternative fuels with gas turbines is the high cost and fuel requirement for a gas turbine engine for completing these tests. APUs, however, are well suited to perform studies and critical evaluations of alternative fuels for use in gas turbines.
This paper reports the results of an experimental campaign to evaluate the gaseous and PM emissions characteristics of an aircraft APU burning various blends of synthetic fuels with Jet A-1 and several other alternative fuels. The study was partially conducted by the FAA CLEEN project at the University of Sheffield’s Low Carbon Combustion Centre and involved teams from the University of Sheffield, British Airways and Rolls-Royce. Gas phase emissions and PM emissions were measured at the engine exit plane.
2.0 PROPERTIES OF FUELS TESTED
Properties and a list of the fuels tested in this study are presented in Table 1. The blending was carried out using grade A glassware. The uncertainty in the volumetric blending was calculated to be approximately ±1%. For ease of data representation, the fuels have been named fuels 1 to 6 and fuel A to H as shown in Table 1. Fuels 1 to 4 are different blends of SPK and Baseline Jet A-1, whereas fuels A to D are different ASTM-approved novel jet fuels to be blended with conventional jet fuel sourced from different companies. Fuel 5 is commercially available diesel, and fuels E-H are novel fuels from different sources not currently approved under ASTM.
Table 1 Pertinent properties of fuels used

2.1 Experimental set-up
A re-commissioned APU gas turbine (Honeywell GTCP85 APU engine) has been used in this study to examine the effect of different fuel blends on emissions and performance. Figure 2 shows a schematic of the experimental set-up used in this study. Real-time data acquisition system and control mechanisms have been used for conducting these experiments. Performance parameters including exhaust gas temperatures, bleed air flow, fuel flow, inlet air flow, engine RPM, pressure and temperature at various core locations have been measured and examined. This APU gas turbine has a two-stage centrifugal compressor. The compressed air mixed with fuel and ignited drives a radial inward-flow turbine wheel. The rotating shaft power of the turbine wheel drives the compressor, which also provides bleed air, and generator.

Figure 2. Experimental set-up.

Figure 3. Gas turbine experimental set-up.
The APU control panel is used to change the bleed flow rate to a predefined value for reaching a set operating condition. Figure 3 shows an actual picture of the APU, inlet duct, generator, transducers and bleed valve mounted on the test bed. All the relevant performance parameters are measured with the help of pressure transducers, flow meters and thermocouples fixed on the engine bed and engine. The bleed duct is connected to a variable solenoid-operated valve before the exhaust to control the flow of bleed air, as bleed air is used for loading the APU (changing operating conditions). This APU also houses a 32 Kilowatt (KW) generator, which is conventionally used to control the operating conditions of the APU. The generator on the APU is not able to change the operating conditions of the engine, since power generated by the APU is substantially higher than that of the generator and no noticeable change in fuel flow and exhaust gas temperature is observed when the generator is fully loaded or when the load on the generator load is changed. Calibration of all the transducers used in the experimental set-up has been carried out regularly to maintain measurement accuracy. The operation panel (PCB-based control panel) is used to monitor exhaust gas temperature, engine operating speed (rpm) and bleed flow rate. This is installed to act as a safety control mechanism incase the more complex control software based on LabVIEW stops responding. The operation panel also provides control of fuel solenoid, starter motor and ignition system. The starter motor is used to spool the engine to 50% speed of engine RPM (maximum) before being cut off. The ignition system is cut off when the engine RPM reaches 95% speed of engine RPM. This is done to avoid any damage to the igniter. Boolean data on the status of the ignition, fuel and oil systems are also fed from the APU to the operation panel for the purpose of operator feedback and emergency shutdown.
Continuous sampling and measurement of gaseous emission have been carried out as per Society of Automotive Engineers (SAE) ARP1256D testing standards. The University of Sheffield’s Mobile Emissions Laboratory has been set up to comply with the SAE ARP1256D standard(41), and has been used to take measurements in this study. The same laboratory has also been used for measuring emissions in various other test campaigns available in literature (Reference Lobo, Hagen and Whitefield19,Reference Rye and Wilson27–Reference Ruslan, Ahmed and Khandelwal31) .
A stainless-steel sampling probe is fixed in place with bolts behind the APU for extractive sampling of exhaust gases. The probes themselves do no conform to the previously mentioned ARP standard. A cross-section traverse of the engine exhaust has been done, and it was found that two locations where these probes were placed had the same AFR and PM. The conditioned exhaust sample is drawn through a 1/4 inch heated sample line into the Mobile Emissions Laboratory for analysis. The sample lines are maintained at 160±5°C per ARP1256D(41) with a minimum bend radius of 10× the line diameter. A constant sampling flow rate (and system pressure) was maintained in the gaseous analysis equipment by using a metal bellows pump, with excess sample flow being exhausted through a back-pressure regulator. Gaseous emissions (unburnet hydrocarbon ‘UHC’, Total Hydrocarbon (THC), carbon monoxide ‘CO’ and nitrous oxide ‘NOx’) were measured per ARP1256D(41). A flame ionization detector (FID), namely a Signal 3000HM hydrocarbon analyser, was used to measure unburned hydrocarbons in the exhaust stream. The concentration of CO and CO2 emissions in the exhaust stream was characterized using a Rosemount Binos 1000 analyser. The unit is a dual channel Non-Dispersive Infrared Analyzer (NDIR), the principle of which is based on the absorption of infrared radiation in the CO and CO2 frequency band. In order to reduce the interference effect of water in the exhaust sample, a chiller was installed upstream of the NDIR analyser to dry the sample. Figure 4 shows the location of sampling probes with reference to the exhaust of engine.

Figure 4. Sampling probe.
An Eco Physics chemiluminescence (CLA EL ht) analyser was used to record NOx in this experiment. Oxygen levels in the exhaust sample were also monitored as a redundant measurement to assess data quality. The analysers were zeroed and then spanned using appropriate gas concentrations just prior to the beginning of each experiment, with the zero and span drift established at engine shutdown. The span gas cylinders were accurate to ±2%. Instrument linearity and interference effects were assessed and corrected for as per the aerospace recommended practices (42). The experimental error associated with the measurement of gaseous emissions is estimated to be approximately ±4% of the reading.
A separate sampling probe was fixed in close proximity to the gaseous emissions sampling probe for measurement of particulates by DMS500. Smoke emissions were measured using the gaseous emissions probe. PM size distribution and mass were tested using a DMS 500 supplied by Cambustion. A separate sampling line heated at 160±5°C was used to supply exhaust gases to the DMS 500. For uniformity with gaseous emission sample lines, the line was fabricated with a minimum bend radius of 10× the line diameter in the sampling line for both DMS 500 and gas sampling line to avoid any error in the readings taken. The DMS500 analysers use electrical mobility measurements to produce particle size/number spectra between 5 nanometres and 1,000 nanometres. Since the classification of particles according to their differing electrical mobility takes place in parallel (rather than in series as in a scanning instrument), the DMS series is able to offer the fastest available size/number spectral measurement of its type. The DMS500 uses a high sample flow rate (8 litres per minute) and unique multiple sheath flows in the charger, resulting in low diffusion losses even of small particles. Figure 5 shows the schematic of the particulate measurement instrument principle. Particles are introduced in the instrument from the left hand side of the tube; particulates are provided a charge by a Unipolar Corona Charger and passed through tube of electrometer detectors to detect the size, weight and number of particulate emissions.
Table 2 Details of measurement instruments


Figure 5. Principle of particulate emission measurement instrument (DMS 500)(43).

Figure 6. Actual pictures of Whatman no. 4 filter paper with varying SAE smoke number.
The SAE smoke number was established using a Richard Oliver smoke meter, Whatman no. 4 filter paper and a reflectometer (EEL43M Smokestain Reflectometer, Diffusion Systems Ltd.) per ARP1179c(44). The technique involves passing a set volume of sample through the conditioned filter paper and measuring the change in the absolute reflectance of the filter paper due to the PM collected. Figure 6 shows four different filter papers with varying SAE smoke numbers for representation purpose. Details of measurement instruments deployed in this study are shown in Table 2.
Three different test windows were selected to determine the performance of the APU for a range of conditions. Sampling at the selected test condition was conducted over a six-minute experimental window once the APU had appeared to stabilize. Figure 7 shows the exhaust temperature profile measured at the exit of the gas turbine with time. One test window is at full RPM when the engine is without any load at an EGT of 300±10°C and AFR of 130±2, the second is with the bleed on at EGT of 445±10°C and AFR of 75±2 and the third with the bleed on at EGT of 580±10°C and AFR of 50±2 test windows before returning the engine to a full power condition prior to shut down. Full RPM window is stable when EGT remains constant at 300±5°C for three minutes. The second and third windows are stable when EGT remains constant at 445±10°C and 580±10°C, respectively, in addition to the bleed mass flow for three minutes.

Figure 7. Exhaust temperature profile for illustration of engine test windows.
3.0 RESULTS AND DISCUSSION
Emissions data concentrations have been converted into emission indices to aid in the quantification of emissions per kilogram of fuel burned. The conversion to emission indices is done as per standard calculation methods(Reference Lobo, Hagen and Whitefield19,42) .
3.1 Gaseous emissions analysis
Table 3 shows gaseous emissions data collected at maximum RPM with EGT of 300±10°C and AFR of 130±2 for different fuels. CO2, CO, NOx and NO emissions are represented in the form of an emission index (EI), whereas NO2,THC is represented as PPM.
It is observed that all the fuels give similar range of emissions. Table 4 represents gaseous emissions data collected at EGT of 450±10°C and AFR of 75±2 for different fuels.
Table 5 represents gaseous emissions data collected at EGT of 580±10°C and AFR of 50±2 for different fuels. Gaseous emissions analysis (CO, CO2, NO, NOx and THC) conducted on fuels studied in this work showed similar performance for all fuels and were within the uncertainty of instruments. It can be seen that for all fuels, NOxremains relatively the same hovering around 4 ppm and also does not appear to change with the engine condition. Moreover, it can be seen that THC for Fuel 6 is extremely high with respect to the other fuels in the first two conditions. Even though total NOxremains relatively constant with respect to engine condition, the amount of NO increases with engine condition. The amount of NO2 also increases as engine condition increases. This phenomenon can be attributed to the higher engine peak flame temperatures reached as more power is extracted from the engine.
3.2 Smoke number
SAE smoke numbers for all the fuels tested in this study are presented in Fig. 8. The accepted accuracy of the method is ±3 smoke numbers, and average of three different samples is presented in Fig. 8. It is observed that fuels 1 to 4 produce substantially lower smoke as compared to fuels with Jet A. Fuel 5 has the highest smoke number of all for all three conditions; this can be expected as fuel 5 is diesel and has the highest aromatic content as compared to the other fuels tested in this study. Diesel’s higher smoke results are in line with other published literature. Fuels E and H have the lowest smoke number of all the fuels tested. Fuel A, B, C and D have significantly lower smoke as compared to Jet A-1. It can be observed from the figure that as the third-power condition smoke emissions go down, this could be due to thermophoretic losses.
Table 3 Gaseous emissions data at condition 1

Table 4 Gaseous emissions data at condition 2

Table 5 Gaseous emissions data at condition 3


Figure 8. SAE smoke number distribution for all fuels and conditions. Fuels 1-4 and A-D(45).

Figure 9. Number and size distribution of particulates measured for 1st condition.
3.3 PM emissions
Figure 9 shows size spectral density comparison for first, second and third conditions for all fuels considered in this study. It can be clearly observed from the figure that particulates emissions decrease with a decline in aromatic content from Fuel 1 to 4. This is consistent with the smoke measurements as shown in Fig. 9. Fuels A, B, C and D follow a trend similar to that of Baseline Jet-A1 fuel. Overall, similar trends are observed at engine operating conditions 2 and 3 as shown in Figs. 10 and 11. It is also observed that at condition 1, fuels with higher aromatic content produce substantially larger particulates as compared to fuels with a lower proportion of aromatics.

Figure 10. Number and size distribution of particulates measured for 2nd condition.

Figure 11. Number and size distribution of particulates measured for 3nd condition.
Figure 12 shows the distribution of particulate mass for all the fuels and conditions tested in this study. To calculate the mass of the particulates coming out of the test engine, effective density of baseline Jet A-1 fuel was used(Reference Johnson, Olfert, Symonds, Johnson, Rindlisbacher, Swanson, Boies, Thomson, Smallwood, Walters, Sevcenco, Crayford, Dastanpour, Rogak, Durdina, Bahk, Brem and Wang46). It can be clearly observed that particulate mass follows the same trend as particulate numbers when aromatics are decreased from Fuel 1 to 4 (shown in Figs. 10–12). Fuel 1 and Fuel B have 24.6% and 21.7% aromatic content by weight, respectively, whereas their mass and particulate size distribution differ substantially. Fuel B gave a substantially better performance in terms of particulate mass and size emissions. The probable reason for the reduction in particulate emissions in the case of Fuel B is due to its aromatic content composition. This result is in line with one of our other studies that found that different aromatic composition behaves in a different manner when it interacts with seals and produces different PM (Reference Corporan, Edwards, Shafer, Dewitt, Klingshirn, Zabarnick, West, Striebich, Graham and Klein22,41) . It is to be noted that seals could lead to production of a small number of volatiles in the exhaust and this aspect has not been covered in this study. Decreasing the number of aromatics in the fuel would lead to reduction in energy density per litre of the fuel, which could eventually lead to reduction in range of the aircraft. Not all aromatics have a direct effect on smoke and particulate emissions; it is just that the right aromatic species need to be selected for reduction in particulate emissions and appropriate seal swell.

Figure 12. Particulates measured for fuels at size of ∼27 nm.
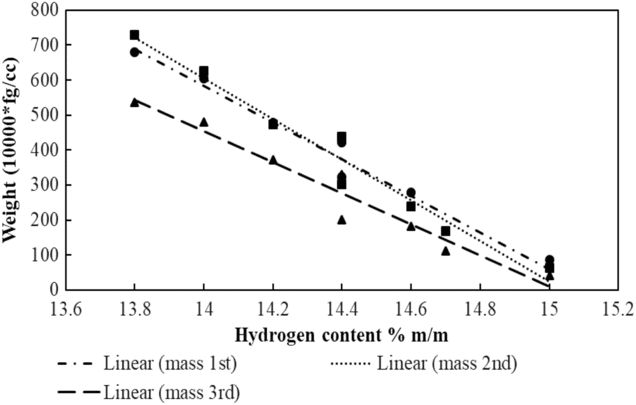
Figure 13. Particulate mass concentration against hydrogen contents of fuels.
Figure 13 above shows the particulate mass concentration against the hydrogen content (mass percentage % m/m). It can be observed from Fig. 13 that the particulate concentration is reduced as the hydrogen content is increased. This can be fundamentally explained by the fact that the more the hydrogen content is, the less the number of carbon-carbon bonds present in the fuel. This observation is in line with other literature such as Christie et al.(Reference Christie, Lobo, Lee and Raper47) and Brem et al.(Reference Brem, Durdina, Siegerist, Beyerle, Bruderer, Rindlisbacher, Rocci-DENIS, Andac, Zelina, Penanhoat and Wang26).
Figure 14 shows the particulate mean gravimetric diameter against the hydrogen content (mass percentage % m/m). It can be observed from the figure that the gravimetric diameter of particulates is reduced as the hydrogen content is increased. These findings are corroborated in studies by Christie et al.(Reference Christie, Lobo, Lee and Raper47) and Brem et al.(Reference Brem, Durdina, Siegerist, Beyerle, Bruderer, Rindlisbacher, Rocci-DENIS, Andac, Zelina, Penanhoat and Wang26).
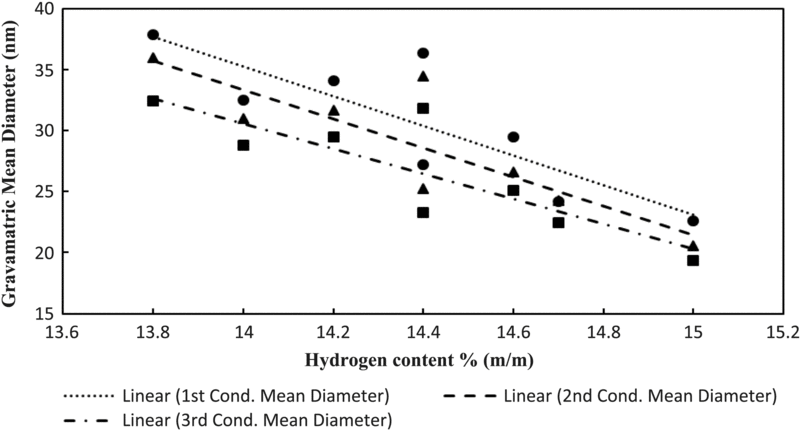
Figure 14. Particulate mean gravimetric diameter against hydrogen content of fuels tested.
4.0 CONCLUSIONS
Gaseous emissions analysis (CO, CO2, NO, NOx and THC) conducted on fuels studied in this paper showed similar performance and results were within the uncertainty of instruments. The smoke and particulate emissions of the SPK fuel were substantially lower than other fuels tested in this study. It is observed from the results that smoke and particulate emissions are decreased with a reduction in aromatic content of the fuel. It has also been observed that PM emissions also depend on the hydrogen content of the fuel. Particulate mass emissions of Fuel B are substantially lower than other comparative fuels, due to different types of aromatics, even though fuel B contains more aromatic content by volume than Fuel A. Further study is required to evaluate the effect of different types of aromatics on particulate emissions. Some investigation into the composition of aromatics and their specific impact on emissions has been studied by Corporan et al.(Reference Corporan, Edwards, Shafer, Dewitt, Klingshirn, Zabarnick, West, Striebich, Graham and Klein22).
ACKNOWLEDGEMENTS
The Honeywell GTCP85 APU used in this test campaign was kindly donated by British Airways. Part of this work was funded by the US Federal Aviation Administration (FAA) Office of Environment and Energy as a part of CLEEN Program under FAA Award Number: DTFAWA-10-C-00006. Any opinions, findings, and conclusions or recommendations expressed in this material are those of the authors and do not necessarily reflect the views of the FAA or other CLEEN Sponsors.