Significant outcomes
-
∙ In total, 12.8% of all tested animals suffered from spinal fractures.
-
∙ Spinal fracture incidence increased over time.
-
∙ X-ray analyses revealed that some animals displayed scapular fractures.
-
∙ High body weight is potentially a risk factor for electroconvulsive seizures (ECS)-induced injuries.
Limitations
-
∙ Only animals from studies 23 and 24 underwent X-ray examination and no X-ray examinations were performed before the ECS treatment.
-
∙ Body weights were only recorded daily for studies 22–24.
Introduction
In the early 1930s, the Hungarian psychiatrist Ladislas von Meduna began experimenting with camphor and other pro-convulsive drugs, in order to induce grand mal epileptic seizures in psychotic patients with catatonic symptoms. These studies were based on the observation that epilepsy was associated with an increased number of glial cells, whereas the opposite applied for schizophrenia, and that the symptoms of schizophrenia were reduced when patients developed epilepsy (Reference Beyer1).
The procedure with chemically induced seizures was associated with severe adverse effects, and in 1938, the Italian neurologist Ugo Cerletti and his colleague, psychiatrist Lucio Bini, developed an improved method where grand mal epileptic seizures were induced electrically via electrodes attached to the patient’s head. This procedure is commonly known as electroconvulsive therapy (ECT) (Reference Beyer1–Reference Shorter and Healy3). ECT is one of the most effective treatments for severe major depression (Reference Pagnin, de Queiroz, Pini and Cassano4), with the highest remission and response rates of all clinically established antidepressant treatments (5).
Initially, ECT was given without muscle relaxation. A rare, but major problem with this unmodified ECT was skeletal injuries, such as spine and hip fractures, and fractures to the long bones (Reference Lingley and Robbins6–Reference Mankad and Weiner8). These fractures were believed to occur due to intense muscle contraction during the seizures (Reference Shaner9). In order to improve the procedure, patients were anaesthetised and given the muscle relaxant agent curare. A safer synthetic muscle relaxant (succinylcholine) was introduced in 1951 (Reference Holmberg and Thesleff10). This improved technique with adequate anaesthesia and muscle relaxation is known as modified ECT.
The ECT procedure has been further refined over the years (regarding electrode placement and stimulation parameters), with the aim of developing a safe method with as few adverse effects as possible [for review see (Reference Fink11)]. Today, patients undergoing ECT are routinely hyperoxygenated, anaesthetised, and administered a muscle relaxant. Since the introduction of the modified ECT procedure, the risk of fractures has decreased, and these are very rare today (Reference Fink12).
Several brain areas are believed to be important for the mode of action of ECT, and different theories behind the antidepressant effect have emerged over the years [for review see (Reference Bolwig13)]. For example, it has been demonstrated that sub-convulsive seizures have inadequate antidepressant effect and that seizures need to be fully generalised in order to be effective (Reference Ottosson14).
ECS is a widely used animal model of ECT that has been extensively studied both in terms of adverse effects and mechanism(s) of action. Several anaesthetics have been applied with ECS, including propofol (Reference Luo, Min and Wei15), ketamine (Reference Stewart and Reid16), carbon dioxide (Reference Galluscio17), and halothane (Reference Bowdler, Green, Minchin and Nutt18). However, it might be difficult to reach the same level of anaesthesia between animals, leading to variation in both seizure threshold and quality of seizures, as these parameters are difficult to evaluate in anaesthetised animals. Consequently, the number of animals in the groups would have to be increased in order to reach sufficient statistical power.
As the application of the electrical current itself serves as an instantaneous anaesthetic, ECS is given without anaesthesia. In this paper, we call this ECS method (without the use of either anaesthesia or muscle relaxation) ‘unmodified ECS’.
Electroconvulsive treatment is in some cases associated with memory disturbances, such as anterograde and retrograde amnesia (Reference Semkovska and McLoughlin19,Reference Lisanby20), and it has been suggested that the use of anaesthetics might affect these cognitive deficits (Reference Miller, Faber, Hatch and Alexander21,Reference Royse, Andrews and Newman22). The use of anaesthetics could also affect behavioural outcomes, such as antidepressant effect (Reference Berman, Cappiello and Anand23). The administration of ECS without anaesthesia can therefore be beneficial in order to separate the effects of the treatment itself from that of the anaesthetic drug.
One major problem with unmodified ECS is that some animals suffer seizure-induced spinal fractures. This leads to paralysis of the hind legs and the animals have to be euthanised. Other types of skeletal damage may also occur, which might affect the behavioural outcome. We have noticed a tendency for increased incidence of spinal fractures in our laboratories over the years, and in a recent experiment, as many as 50% of the animals suffered spinal fractures, highlighting the extent of this problem. Surprisingly, this issue has only been addressed sporadically in the literature.
Since the introduction of the 3R principles in 1959 (Reference Russell and Burch24), researchers have strived towards fulfilling the criteria of replacement, reduction, and refinement. If replacement of the use of animals within research is not an option, then group sizes should be reduced to the smallest possible (often based on power calculations) and the techniques refined, so the animals experience as little suffering and pain as possible. The high incidence of fractures and subsequent exclusion of animals from the studies presented here means that larger group sizes are needed and that there is a risk for a higher degree of suffering as well as for skewing of results due to pre-selected study groups. Such experiments are neither in compliance with best practice and high translatability nor the reduction and refinement principles of the 3Rs. Herein we highlight the problem of fractures in ECS models, discuss matters and take both refinement, reduction, and translatability into consideration – finally suggesting potential solutions to how to approach the challenges in this model.
Aim of the study
In this article, we summarise our findings on unmodified auricular ECS and fractures in different rat strains, and discuss possible explanations, with suggestions for future studies.
Materials and methods
This article summarises 24 studies conducted during 2009–2015. We have included studies in which animals (514 rats in total) received (or were planned to receive) a series of five or eight consecutive ECS treatments (one treatment per day). Table 1 presents a summary of study ID, year, rat strain, breeder/provider, concomitant treatment (including number of animals receiving the treatment), body weight (at the beginning of the studies), ECS apparatus, ECS parameters, number of consecutive ECS treatments, number of animals receiving a series of ECS, and number and percentage of animals suffering from fractures. All animals in this summary received auricular ECS. Only male animals were used, and experiments 1–21 were carried out according to guidelines set by the Malmö-Lund Ethical Committee for the use and care of laboratory animals. For experiments 22–24, the Danish Committee on Ethics in Animal Experimentation approved the study protocols.
Table 1 Overview of the studies in this review
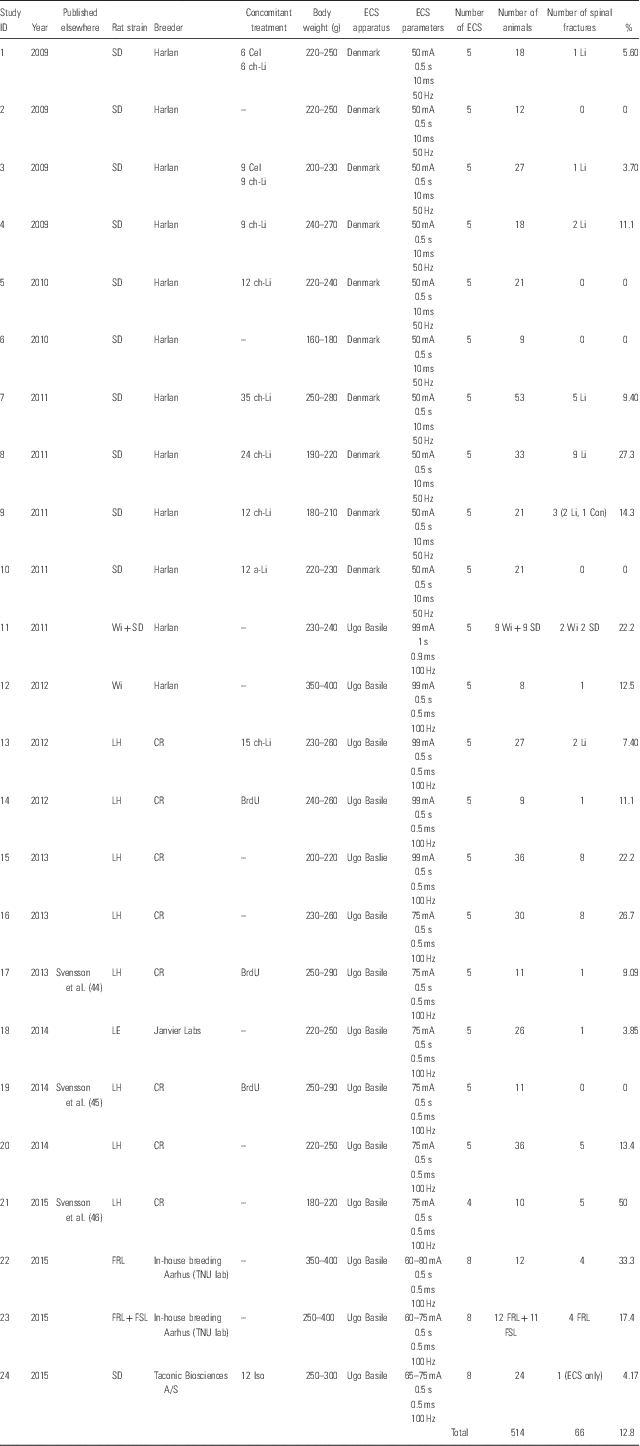
a-Li, acute lithium treatment [injected intraperitoneally 8 h before each electroconvulsive seizures (ECS), 4 mEq/kg rat]; BrdU, 5-bromo-2’-deoxyuridine (injected intraperitoneally twice daily, 50 mg/kg rat); Cel, celecoxib treatment (administered via chow, 15 or 50 mg/kg rat); ch-Li, chronic lithium treatment (administered via chow, 2 g lithium chloride/kg rat); Con, control animal; CR, Charles River; FRL, Flinders-resistant line; FSL, Flinders-sensitive line; Iso, isoflurane inhalation anaesthesia [300 ml/min, 5% until interdigital reflex was absent (after ~2 min)]; LE, Long Evans; LH, Lister Hooded; SD, Sprague Dawley; TNU, Translational Neuropsychiatry Unit; Wi, Wistar.
ECS
One of two ECS apparatuses (a pulse generator manufactured in Denmark or the 57800–01 ECT Unit, Ugo Basile, Gemonio, Italy) was used to generate electrical current delivered via ear clip electrodes (Somedic Sales AB, Solna, Sweden or Ugo Basile, Gemonio, Italy). The Danish pulse generator was used in studies 1–10, and the Italian unit was used in studies 11–24. One daily ECS treatment was given for 5 consecutive days in all studies except in study 21 (where ECS was given for 4 consecutive days) and studies 22–24 (where ECS was given for 8 consecutive days). The interval between consecutive treatments was 24 h in all experiments. An overview of stimulus parameters for the studies is presented in Table 1. In studies 22–24, the current ranged between 60 and 80 mA depending on the individual size of the rat as well as strain, and was chosen to ensure generalised seizures. During the ECS procedure, the experimenter held the animal in an upright (vertical) position, gently pressed against the experimenter’s chest. The ear clip electrodes were then carefully placed on the animal’s ears. Immediately after electrical stimulation, the rat was removed from the experimenter’s chest (but still held in one hand) so that its body was allowed to move freely.
Radiography
To evaluate skeletal injuries, animals in studies 23–24 were X-rayed (Eklin Mark III™ portable digital radiography system, Sound-Eklin, Carlsbad, CA, USA). In study 23, animals displaying injury were X-rayed (n=3). In study 24, all animals receiving ECS treatment were X-rayed (n=24) at the end of the experiment. Exposure factors were 50 kV and 0.5 mAs.
Statistical analyses
The percentage of animals suffering from spinal fractures in relation to all ECS-treated animals was calculated per study. The percentage of animals suffering from spinal fractures was also calculated per strain. A Spearman’s rank-order correlation was used to analyse the association between ranked percentages of spinal fractures and time. Fisher’s exact test was used to analyse the association between strain and number of spinal fractures. Body weights were compared using Student’s t-test. GraphPad Software, Prism 6 and IBM SPSS Statistics 23 were used for calculations and graphs.
Results
An overview of the studies in this review is provided in Table 1.
Spinal fractures
The signs associated with spinal fractures were similar for all animals regardless of rat strain. When the current is applied, an immediate faint cracking sound can sometimes be heard, followed by rotation of tail and hind legs. When this happens, the tonic phase is absent and an intense clonic phase often follows the rotation. After the intervention, the animal displays paralysis of both hind legs (paraplegia).
In total, 12.8% (66 out of 514) of the animals suffered spinal fractures. The weight of these animals ranged between 190 and 420 g. Table 1 summarises the percentages of spinal fractures per study, and the total percentage of spinal fractures for all 24 studies. Table 2 shows that the occurrence of spinal fractures was not rat strain specific, as animals from five out of six strains displayed paraplegia after ECS. However, Fisher’s exact test revealed a strong association between strain and number of fractures (p=0.002). From Table 2 we can conclude that the risk of developing fractures was highest in Flinders-resistant line (FRL, 33.3%), Lister Hooded (LH, 17.6%), and Wistar (Wi, 17.6%). Fig. 1a displays the percentages of animals suffering spinal fractures in the studies in chronological order. Spearman’s rank-order correlation revealed an increase in spinal fracture incidence over time (r s (22)=0.403, p=0.05). Figure 1b shows the number of spinal fractures per treatment. The incidence of spinal fractures was highest during the second and third treatment.
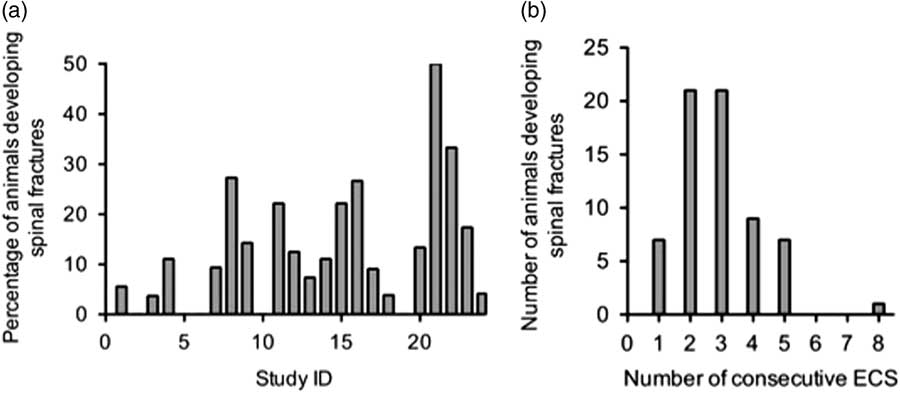
Fig. 1 (a) Chronological order of studies 1–24 and the percentages of animals developing spinal fractures. (b) Number of spinal fractures per treatment. Note that only animals in studies 22–24 received eight electroconvulsive seizures (ECS) treatments in total, as compared with a total of five treatments in studies 1–21. In this summary, most animals developed spinal fractures during the second and third ECS treatment.
Table 2 Number of spinal fractures per rat strain

Spinal ‘cracking’
We observed that when some animals receive ECS, a cracking or popping sound originating from the spinal column is produced. This cracking can both be heard and felt by the experimenter. Notes about these cracking sounds were recorded in studies 13–21 (LH and Long Evans (LE) rats; 196 animals in total) to examine whether spinal cracking could potentially predict spinal fractures. In all, 71 cracking sounds were noted in a total of 57 animals. In total, 31 animals suffered from spinal fractures in these studies, and we noted cracking sounds during an earlier treatment in ten of these 31 animals (32.3%).
Skeletal injuries confirmed with X-ray analysis
We observed that some animals showed restricted movement of the front legs following ECS treatment. In study 23, three animals showed this behaviour and they were therefore euthanised and subsequently X-rayed. One animal (FRL) displayed spinal fracture, and the remaining two animals (one Flinders-sensitive line and one FRL) had scapular fractures (fractures to the shoulder blades, Figs 2a and b). In study 24, X-ray analysis confirmed a spinal fracture in one animal (treated with ECS only, Fig. 2c). An additional animal in study 24 (treated with ECS and isoflurane) showed restricted movement of the front legs, but no fractures were detected by X-ray. All rats in study 24 were X-rayed, and no other animal had fractures. To date, we do not have data indicating the frequency of scapular fractures.

Fig. 2 X-ray images of scapular fractures in a Flinders-sensitive line (FSL) rat (a) and a Flinders-resistant line (FRL) rat (b). Spinal fracture of a Sprague Dawley (SD) rat (c). Fractures are indicated by white arrows.
In studies 22–24, body weight was recorded daily and the results suggest that injured animals were slightly larger than non-injured animals (t(57)=2.126, p<0.05, Fig. 3).
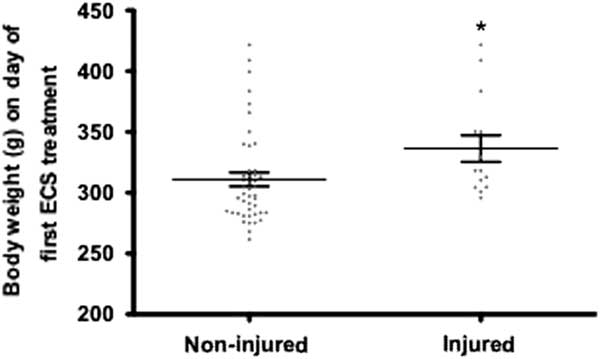
Fig. 3 Body weights of all electroconvulsive seizures (ECS)-treated animals from experiments 22–24. Injured animals (spinal and scapular fractures) had a slightly higher body weight compared with non-injured rats. *p<0.05.
Discussion
An average of 12.8% of the animals undergoing unmodified ECS treatment from the 24 studies presented here suffered spinal fractures. To compensate for this, more animals had to be included in the ECS groups, and in some cases studies have had to be aborted prematurely. For example, the reason for giving four ECS treatments in study 21 was that the incidence of fractures during ECS was exceptionally high (50% of the animals suffered spinal fractures). Among the 24 studies included in this summary, we noted an intra-strain difference in the incidence of fractures regarding the Sprague Dawley (SD) strain. In some of the earlier studies, no animals suffered spinal fractures. Interestingly, the frequency of ECS-induced spinal fractures has increased over time (Fig. 1a). Possible explanations for this increase and suggestions for solutions are discussed below.
Summary of studies on ECS-induced fractures
ECS-induced spinal fractures have been described by several researchers over the years (Reference Hayes25–Reference Andrade, Shaikh, Narayan, Blasey and Belanoff29). In an early study by Hayes (Reference Hayes25), 4.5% (five out of 110) of the rats displayed one- or two-leg (paraplegic) paralysis after ECS. Post-mortem examinations of two paraplegic animals revealed badly fractured vertebrae. The risk of injury was not related to electric current intensity. Interestingly, a relationship between body weight and frequency of injuries was found in studies 22–24 (Fig. 3), with heavier animals being at greater risk. Increased body weight as a risk factor has also been suggested by other authors (Reference Stern, McDonald and Werboff26,Reference Andrade, Sudha and Venkataraman30), and in one study it was reported that a reduction of body weight (by water deprivation) reduced the incidence of fractures during ECS (Reference McDonald31). A frequently applied animal model of epilepsy and a screening test for anticonvulsant drugs, the maximal electroshock seizure (MES) is used to induce grand mal epileptic seizures in mice [for review see (Reference Löscher32)]. No studies reporting skeletal injuries during ECS or MES in mice were found. The body weight of mice is substantially lower than rats’, which could support the potential relationship between heavier animals being at greater risk for developing fractures after the induction of grand mal epileptic seizures. It has though also been reported that the risk of fractures not only applies to older and larger animals with greater muscle mass, but also to very young animals (Reference Andrade, Sudha and Venkataraman30). In the present survey, the weights of the animals that suffered spinal fractures ranged between 190 and 420 g. This large range, in addition to missing data regarding exact body weight at the time of fractures for studies 1–21, makes it difficult to draw any final conclusions about the relationship between weight and fracture incidence.
In a study by Stern et al. (Reference Stern, McDonald and Werboff26), it was reported that 54% of the animals (SD rats) had to be euthanised due to ECS-induced spinal fractures. The risk of developing fractures decreased after the third treatment (in a series of 15 treatments), and the incidence of fractures was highest during the second treatment. This is in line with our observations, where the incidence of spinal fractures was highest during the second and third treatment (Fig. 1b).
Skeletal injuries confirmed with X-ray analysis
Apart from spinal injuries, we found that some animals displayed scapular fractures (Figs 2a and b). Although these fractures were detected in animals that displayed restricted movement of their front leg after ECS, we cannot rule out that they did not have some skeletal damage before the treatment, as X-ray analysis was only performed after the experiment. Although unlikely, it cannot be ruled out that the animals had acquired scapular injuries during breeding or during transportation from the breeder to the animal facility. However, spinal injuries were also observed both in animals from a commercial breeding facility and in animals that were bred in-house. In study 24, all animals were thoroughly examined before ECS and no behavioural abnormalities were found, making it unlikely that pre-ECS skeletal injuries were present.
One concern is that ECS-induced skeletal injuries may also be present in some animals without visible impairment of front leg movement, and might, if associated with pain, affect the outcome in behavioural studies. Prey animals seldom express signs of pain, and symptoms of injuries may therefore be less apparent and difficult to detect. It has been suggested that some skeletal injuries associated with unmodified clinical ECT are symptomless (Reference Dewald, Margolis and Weiner7,Reference Epstein33). Animals experiencing severe pain may cease to groom, display hypoactivity, and weight loss. We have noted that the body weight of animals receiving ECS treatment stagnate over the treatment course. This could potentially be related to discrete injuries, such as scapular fractures. Even though we have not observed that ECS-treated animals cease to groom or show other apparent signs of pain, it is possible that the injuries affect behaviour. Scapular fractures after epileptic seizures have also been reported in the clinical literature, and some studies report scapular fractures following ECT treatment (Reference Lingley and Robbins6,Reference Ramin and Veit34,Reference Mathews, Cocke and D’Ambrosia35).
Risk factors for ECS-induced fractures
It is generally believed that electroconvulsive treatment-induced fractures occur due to intense muscle contractions (Reference Shaner9), and, as described above, a proposed risk factor is high body weight. It has also been suggested that a high stimulus intensity relative to the seizure threshold might increase the risk of spinal fractures (Reference Andrade, Thyagarajan, Vinod, Srikanth, Rao and Chandra27). We have applied several combinations of treatment parameters (current intensity, duration, pulse width, frequency; see Table 1) throughout the years in an attempt to reduce the fracture incidence, but without success. Inbreeding [which might decrease bone strength (Reference Rudan, Skarić-Jurić and Smolej-Narancić36)] is another possible risk factor, and hip fractures during ECT have been linked to osteoporosis (Reference Nott and Watts37). Some of our animals may have had osteoporosis or other defects in bone development and density. The fact that spinal fractures occurred in almost all reviewed rat strains might contradict this explanation. However, we did find that some strains (FRL, LH, Wi) were more prone to develop fractures than others (as indicated by the significant association between strain and number of spinal fractures, p=0.002), and we did observe an increased incidence over time (p=0.05). This increased incidence over time could reflect changes in breeding strategies, as the animals appear to have become more fragile over time. Furthermore, data from studies 22–24 suggest a potential relationship between body weight and frequency of injuries, with heavier animals being at greater risk. From Table 2, we can conclude that SD and LE rats appear most suitable for receiving auricular ECS, a finding that should be considered when designing future studies.
We have not been able to explain the spinal fractures by other treatments concomitant to ECS. For example, both lithium-treated and control animals displayed ECS-induced fractures (Table 1). We have used two different models of ECS apparatuses and also replaced the ear clip electrodes frequently several times. These factors did not appear to affect the incidence of fractures. Individual differences in animal handling during the ECS procedure did not affect the incidence, since all experimenters over the years have observed spinal fractures.
Can ECS-induced fractures be prevented and thus improve translatability and the 3Rs?
Fractures may be inevitable in unmodified ECS, but the use of anaesthesia and muscle relaxation may decrease the incidence. The use of anaesthesia and muscle relaxation might not only decrease the risk of fractures, but also substantially increase the translational value of the model. However, depending on the specific research aims, it is not always feasible to implement a modified ECS method, as the results may become inconsistent. It has been suggested that the use of anaesthetic agents affect the cognitive deficits after electroconvulsive treatment and that these agents might have anticonvulsant effects (Reference Miller, Faber, Hatch and Alexander21,Reference Andrade, Sudha and Venkataraman30,Reference Fochtmann38,Reference Seshadri and Mazi-Kotwal39). The use of anaesthesia has also been reported to preserve some memory functions when administered before/during ECS (Reference Luo, Min and Wei15,Reference Fochtmann38,Reference McDaniel, Sahota, Vyas, Laguerta, Hategan and Oswald40). Since our main research aim has been to investigate ECS-associated cognitive impairment, it has been important to reduce the number of confounding factors. It is also difficult to confirm that ECS treatment elicits generalised (tonic–clonic) seizures when muscle relaxants are used.
In our more recent studies (studies 13–21) we have noted spinal cracking sounds during ECS. These cracking sounds were only noticed in 32.3% of the animals that subsequently developed spinal fractures. Furthermore, many animals made these cracking sounds without developing symptoms of spinal fractures. Cracking sounds therefore do not appear to predict spinal fractures.
The need for developing new, as well as improve traditional, animal models with better construct, face, and predictive validity has recently been emphasised (Reference Stewart and Kalueff41,Reference Nestler and Hyman42). Indeed, the standard auricular ECS method differs from clinical settings in several ways, for example, electrode placement (and hence seizure generalisation) and use of anaesthesia and muscle relaxation. In an attempt to closely mimic the clinical ECT procedure, a new animal model has recently been developed, where ECS is administered via screw electrodes implanted in the skull close to the motor cortex (to resemble the clinically used electrode placement) (Reference Theilmann, Löscher, Socala, Frieling, Bleich and Brandt43). It was found that animals receiving standard auricular ECS showed greater signs of fear during the treatment sessions compared with animals receiving cortical ECS, suggesting that unmodified auricular ECS might not be the ideal ECT model.
We suggest, whenever possible, to routinely evaluate locomotor activity and grip strength before and after ECS treatment. Furthermore, body weights should be monitored closely and a thorough physical examination of the animals before and after each ECS session should be performed. This would hopefully detect subtle skeletal injuries that do not cause substantial mobility impairments or paralysis.
We hope that this summary has shed light on an important issue with the ECS procedure currently used in many research laboratories. This problem not only introduces potential confounding factors that can affect the interpretation of results and challenge the translatability of the preclinical studies, but also conflicts with the 3R guidelines of animal experiments. The 3Rs, first introduced in 1959 by Russell and Burch (24) are becoming a more and more integrated part of good research practice. Apart from replacement of animal experiments, focus is often on the reduction to spare the number of animals used. A thorough look on the refinement is, however, warranted in this model since this focus may – apart from reducing the suffering of the animals – improve the model’s validity per se. In most cases the possible confounding effect of anaesthesia is preferred to the skewing of results that a pre-selected study group may lead to. It is still not clear which approach will lead to the use of fewest animals. However, for now, the use of anaesthesia will most certainly fulfil the 3Rs to a higher degree than circumventing the use of anaesthesia. In order to keep refining this model, we strongly encourage attempts to develop improved procedures where the problem of skeletal damage is avoided without introducing factors that increase variability.
Acknowledgements
The radiography system was kindly provided by Frederik Nielsen. Authors’ Contributions: M.E. has contributed to study design, data collection, and data analyses as well as drafting of the manuscript. M.K.J. has contributed to study design, data collection, and data analysis as well as critical revision of the manuscript. M.G. has contributed by collecting and analysing data. A.T. has contributed to study design, data analyses, and interpretation of the data. B.K. has contributed to interpretation of data and revision of the manuscript. G.W. has contributed to study design and interpretation of data. C.B.-R. has contributed to study design, data collection, data analysis, and data interpretation as well as critical revision of the manuscript. All authors met the following authorship criteria: (1) substantial contributions to conception and design of, or acquisition of data or analysis and interpretation of data, (2) drafting the article or revising it critically for important intellectual content, and (3) final approval of the version to be published.
Financial Support
This research was funded by Central Region Denmark’s Psychiatric Research Foundation, The Swedish Research Council, The Royal Physiographic Society of Lund, Greta och Johan Kocks Stiftelser, Stiftelsen Professor Bror Gadelius Minnesfond, and OM Persson Foundation. The funding sources had no role in the design and conduct of the study; collection, management, analysis, and interpretation of the data; preparation, review, or approval of the manuscript; and decision to submit the manuscript for publication.
Conflicts of Interest
G.W. is Editor-in-Chief and C.B.-R. is Associate Editor of Acta Neuropsychiatrica, but they were not involved and actively withdrew during the review and decision process of this manuscript.
Animal Welfare
This study is in compliance with the ARRIVE (Animal Research Reporting In Vivo Experiments) guidelines.
Ethical Standards
The authors assert that all procedures contributing to this work comply with the ethical standards of the relevant national and institutional guides on the care and use of laboratory animals.