Book contents
- Fetal and Neonatal Brain Injury
- Fetal and Neonatal Brain Injury
- Copyright page
- Contents
- Contributors
- Preface
- Section 1 Epidemiology, Pathophysiology, and Pathogenesis of Fetal and Neonatal Brain Injury
- Chapter 1 Neonatal Encephalopathy
- Chapter 2 Neuronal Cell Death Mechanisms Relevant to Humans
- Chapter 3 Cellular and Molecular Biology of Hypoxic-Ischemic Encephalopathy
- Chapter 4 The Pathogenesis of Preterm Brain Injury
- Section 2 Pregnancy, Labor, and Delivery Complications Causing Brain Injury
- Section 3 Diagnosis of the Infant with Brain Injury
- Section 4 Specific Conditions Associated with Fetal and Neonatal Brain Injury
- Index
- References
Chapter 2 - Neuronal Cell Death Mechanisms Relevant to Humans
Rethinking Modeling Neonatal Hypoxic-Ischemic Encephalopathy
from Section 1 - Epidemiology, Pathophysiology, and Pathogenesis of Fetal and Neonatal Brain Injury
Published online by Cambridge University Press: 13 December 2017
- Fetal and Neonatal Brain Injury
- Fetal and Neonatal Brain Injury
- Copyright page
- Contents
- Contributors
- Preface
- Section 1 Epidemiology, Pathophysiology, and Pathogenesis of Fetal and Neonatal Brain Injury
- Chapter 1 Neonatal Encephalopathy
- Chapter 2 Neuronal Cell Death Mechanisms Relevant to Humans
- Chapter 3 Cellular and Molecular Biology of Hypoxic-Ischemic Encephalopathy
- Chapter 4 The Pathogenesis of Preterm Brain Injury
- Section 2 Pregnancy, Labor, and Delivery Complications Causing Brain Injury
- Section 3 Diagnosis of the Infant with Brain Injury
- Section 4 Specific Conditions Associated with Fetal and Neonatal Brain Injury
- Index
- References
Summary
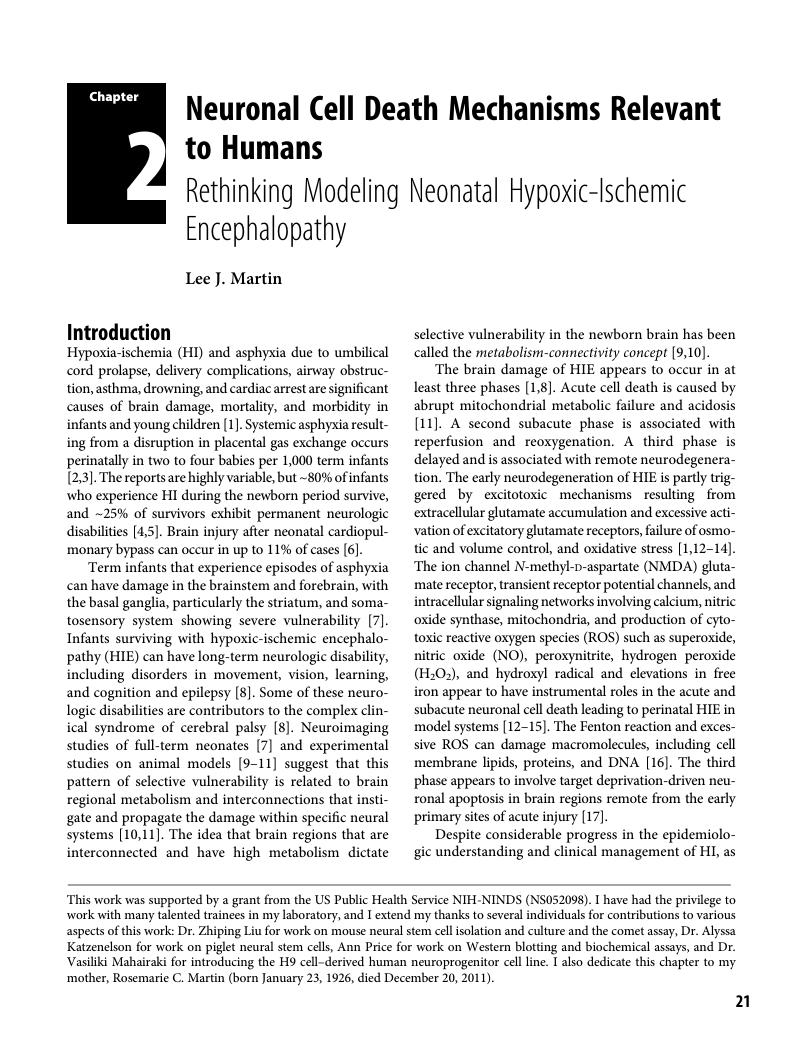
- Type
- Chapter
- Information
- Fetal and Neonatal Brain Injury , pp. 21 - 35Publisher: Cambridge University PressPrint publication year: 2017