1. Introduction
Oceanic anoxic events (OAEs) represent crucial episodes during the history of the Earth because the associated climatic, palaeoceanographic and geochemical changes account for events including biotic crises and mass extinctions (e.g. Meyer & Kump, Reference Meyer and Kump2008; Jenkyns, Reference Jenkyns2010). The mid-Tournaisian event, which occurred during the Mississippian (355 Ma), is among the least studied OAE (Siegmund et al. Reference Siegmund, Trappe and Oschmann2002; Bábek et al. Reference Bábek, Kumpan, Kalvoda and Grygar2016; Rakociński et al. Reference Rakociński, Marynowski, Zatoń and Filipiak2021 a, Reference Rakociński, Książak, Pisarzowska and Marynowski2022). The global transgression during the mid-Tournaisian is associated with anoxic conditions during deposition of sediments in many parts of the world (e.g. Siegmund et al. Reference Siegmund, Trappe and Oschmann2002; Bábek et al. Reference Bábek, Kumpan, Kalvoda and Grygar2016; Rakociński et al. Reference Rakociński, Marynowski, Zatoń and Filipiak2021 a, Reference Rakociński, Książak, Pisarzowska and Marynowski2022), and this event is also termed the Lower Alum Shale Event (LASE; see Becker, Reference Becker and House1993 a,b; Walliser, Reference Walliser and Walliser1996; Becker et al. Reference Becker, Kaiser, Aretz, Becker, Königshof and Brett2016). The LASE was characterized by distinctive facies changes in deep portions of open-marine environments including the transformation from pelagic carbonate sedimentation to the deposition of organic-rich black shales and cherts/radiolarites or black to dark grey radiolarian cherts and lydites (e.g. Siegmund et al. Reference Siegmund, Trappe and Oschmann2002; Bábek et al. Reference Bábek, Kumpan, Kalvoda and Grygar2016; Rakociński et al. Reference Rakociński, Marynowski, Zatoń and Filipiak2021 a, Reference Rakociński, Książak, Pisarzowska and Marynowski2022). These changes affected pelagic and benthic organisms, such as goniatites, brachiopods, gastropods, bivalves and trilobites (see e.g. Becker, Reference Becker and House1993 a; Walliser, Reference Walliser and Walliser1996; Kaiser et al. Reference Kaiser, Becker, Steuber and Aboussalam2011). The association between the LASE and volcanic activities caused climatic changes, which increased marine productivity and simultaneously stopped carbonate production (e.g. Siegmund et al. Reference Siegmund, Trappe and Oschmann2002; Rakociński et al. Reference Rakociński, Marynowski, Zatoń and Filipiak2021 a, Reference Rakociński, Książak, Pisarzowska and Marynowski2022). The LASE preceded the climatic cooling and globally marked positive carbon isotope shift in the mid-Tournaisian that is known as the mid-Tournaisian carbon isotope excursion (TICE) or Kinderhookian–Osagean δ13C excursion (e.g. Buggisch et al. Reference Buggisch, Joachimski, Sevastopulo and Morrow2008; Chen et al. Reference Chen, Chen, Qie, Huang, He, Joachimski, Regelous, Pogge von Strandmann, Liu, Wang, Montañez and Algeo2021). However, knowledge on this biotic turnover remains scant, especially relative to geochemical changes in palaeoenvironments at a supra-regional scale (see Rakociński et al. Reference Rakociński, Marynowski, Zatoń and Filipiak2021 a, Reference Rakociński, Książak, Pisarzowska and Marynowski2022).
Therefore, in the present study, high-resolution inorganic geochemistry and framboidal pyrite analyses were used to investigate changes in depositional conditions during the mid-Tournaisian anoxic event in the Montagne Noire region, France. The present study revealed that in the Montagne Noire region, the LASE interval is preserved as black radiolarian cherts that are poor in organic content. These sediments exhibit no evidence of continuous anoxic conditions relative to those reported in other parts of the world.
2. Geological setting
During the Late Devonian and Mississippian periods, the present-day Montagne Noire region belonged to the North Gondwana margin (Faure & Ferrière, Reference Faure and Ferrière2022). This was probably a part of the peri-Gondwanan Galatian superterrane (Stampfli et al. Reference Stampfli, Hochard, Vérard, Wilhem and von Raumer2013) or the Armorica microcontinent (Girard et al. Reference Girard, Cornée, Joachimski, Charruault, Dufour and Renaud2020), which were bounded by the Rheic Ocean to the NW and the Palaeotethys to the SE (Fig. 1a). According to palaeogeographic reconstructions, this area migrated from subtropical latitudes towards the equator and Laurussia during the Devonian Period because of the closure of the Rheic Ocean (Franke et al. Reference Franke, Cocks and Torsvik2017). However, the precise palaeogeographic position of this microplate and its relation to the Laurussia continent during middle Palaeozoic time remains debatable (see von Raumer et al. Reference von Raumer, Bussy, Schaltegger, Schulz and Stampfli2013; Eckelmann et al. Reference Eckelmann, Nesbor, Königshof, Linnemann, Hofmann, Lange and Sagawe2014; Franke et al. Reference Franke, Cocks and Torsvik2017).
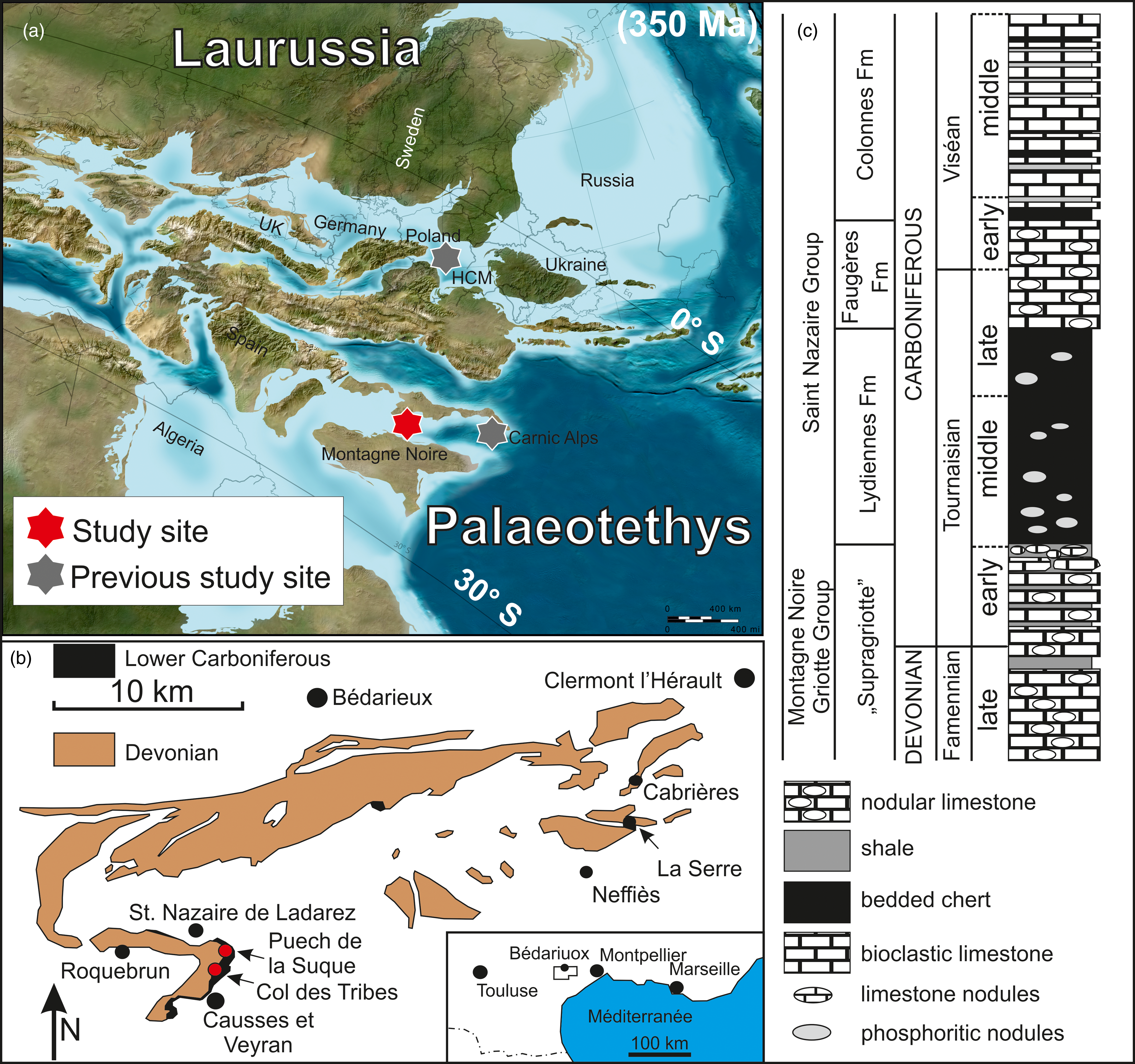
Fig. 1. (a) Palaeogeography of Europe during the Tournaisian Age ∼350 Ma (Blakey, Reference Blakey2012) showing the location of the Montagne Noire area (red star); © 2012 Colorado Plateau Geosystems Inc. (b) Location of the Puech de la Suque and Col des Tribes sections in the southeastern part of the Montagne Noire (Girard, Reference Girard1994, Reference Girard1996). (c) Lithological log (not to scale) of the Mont Peyroux Succession based on outcrops in the Puech de la Suque area (Aretz, Reference Aretz2016). Lithostratigraphy is according to Korn & Feist (Reference Korn and Feist2007). For detail about previous study site see to Rakociński et al. (2021b, 2022).
Upper Devonian to Mississippian sediments in the southeastern portion of the Montagne Noire, southern Massif Central in France, are represented by deep-water pelagic successions in the Mont Peyroux Nappe area and shallow-water successions in the ‘Cabrières Klippen’ area (Flajs & Feist, Reference Flajs and Feist1988; Aretz, Reference Aretz2016; Bábek et al. Reference Bábek, Kumpan, Kalvoda and Grygar2016; Feist et al. Reference Feist, Cornée, Corradini, Hartenfels, Aretz and Girard2021). The studied sections are in the Mont Peyroux Nappe area (Fig. 1b; Kaiser et al. Reference Kaiser, Steuber and Becker2008; Girard et al. Reference Girard, Cornée, Corradini, Fravalo and Feist2014) and these include the inverted Puech de la Suque (PDLS) section (43° 29′ 58.6″ N and 03° 05′ 55.6″ E) on the SE slope of Puech de la Suque hill. This section is c. 2.2 km SE of St Nazaire de Ladarez and c. 15 km SW of Bédarieux (Fig. 1b, online Supplementary Material Figs S1–S2; see Feist et al. Reference Feist, Cornée, Corradini, Hartenfels, Aretz and Girard2021). Relatedly, the inverted Col des Tribes (CDT) section (43° 29′ 23.6″ N and 03° 05′ 27.5″ E) is on the east slope of Mont Peyroux hill, which is c. 2 km north of Causses et Veyran and c. 1.5 km SSW of the PDLS section (Fig. 1b, online Supplementary Material Fig S3; Girard et al. Reference Girard, Cornée, Corradini, Fravalo and Feist2014; Feist et al. Reference Feist, Cornée, Corradini, Hartenfels, Aretz and Girard2021). Both sections represent pelagic facies that contain micritic cephalopod-rich nodular limestones with marly shale intercalations and black to dark grey radiolarian cherts. These sediments involve the following major lithostratigraphic units: (1) the upper Montagne Noire Griotte Group, which encompasses the upper Famennian to lower Tournaisian nodular limestone and shales and (2) the Lydiennes Formation of the Saint Nazaire Group, which contains black and dark grey radiolarian cherts with phosphatic nodules (see Figs 1c, 3; see Korn & Feist, Reference Korn and Feist2007; Aretz, Reference Aretz2016). The black radiolarian cherts in the Lydiennes Formation range in age from ‘mid’ to early late Tournaisian (Figs 1c, 2; for details see e.g. Korn & Feist, Reference Korn and Feist2007). The Lydiennes Formation is overlain by nodular limestones of the Faugères Formation in the Saint Nazaire Group, which are dated as latest Tournaisian to early Visean (Korn & Feist, Reference Korn and Feist2007; Aretz, Reference Aretz2016; Fig. 1c).
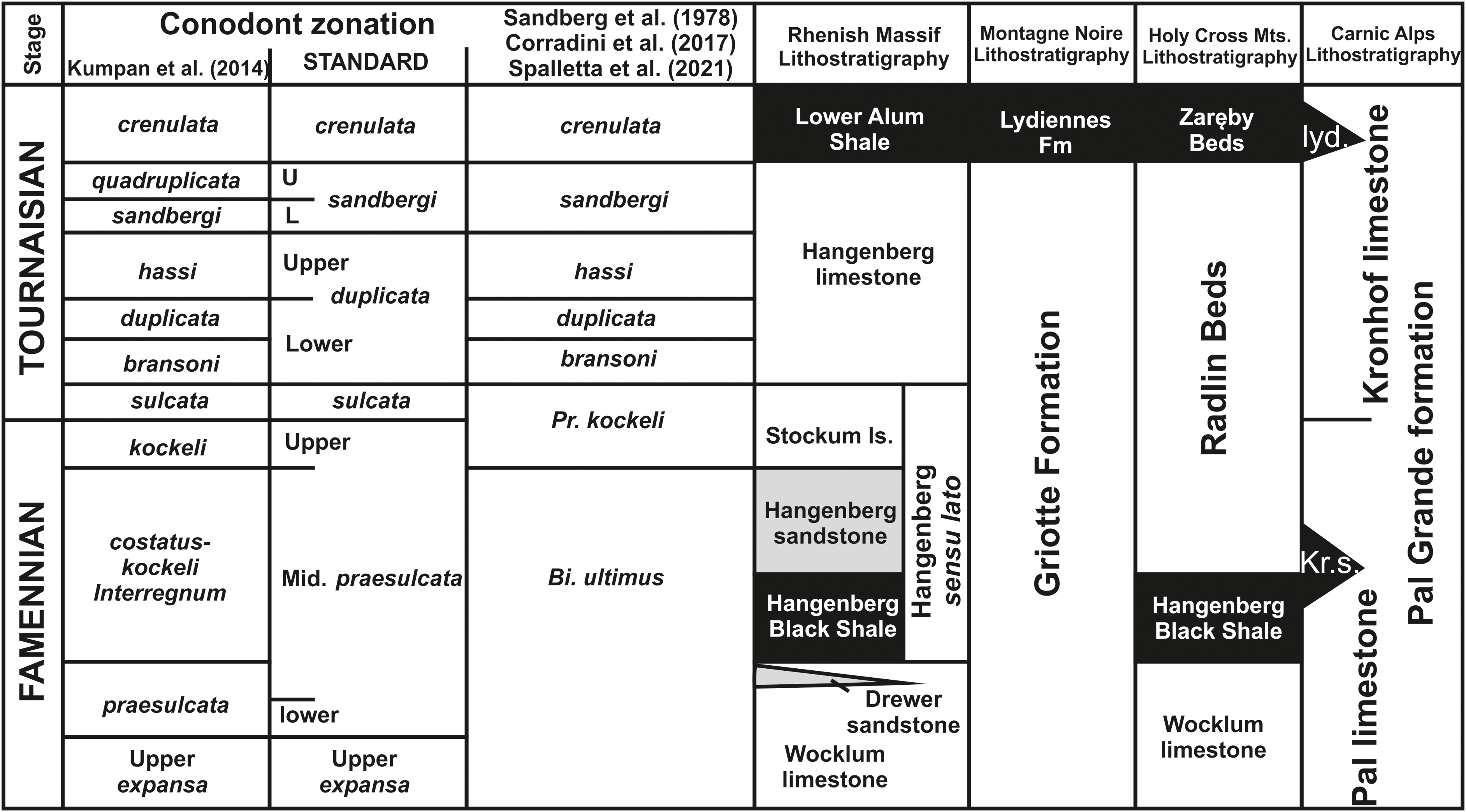
Fig. 2. Conodont zonation and lithostratigraphy for the Montagne Noire in comparison to the Rhenish Massif, Holy Cross Mountains and the Carnic Alps (based on Sandberg et al. Reference Sandberg, Ziegler, Leuteritz and Brill1978; Kumpan et al. Reference Kumpan, Bábek, Kalvoda, Frýda and Matys Grygar2014; Corradini et al. Reference Corradini, Pondrelli, Schönlaub and Suttner2017; Spalletta et al. Reference Spalletta, Corradini, Feist, Korn, Kumpan, Perri, Pondrelli and Venturini2021). Abbreviations: Kr.s. – Kronhof shale (equivalent HBS); ls. – limestone; lyd. – lydite; L – lower; Mid. – middle; U – upper; Bi. – Bispathodus; Pr. – Protognathodus. Lithostratigraphy is based partly on Aretz (Reference Aretz2016), Bábek et al. (Reference Bábek, Kumpan, Kalvoda and Grygar2016), Czarnocki (Reference Czarnocki1989), Malec (Reference Malec2014) and Rakociński et al. (Reference Rakociński, Marynowski, Zatoń and Filipiak2021 a).
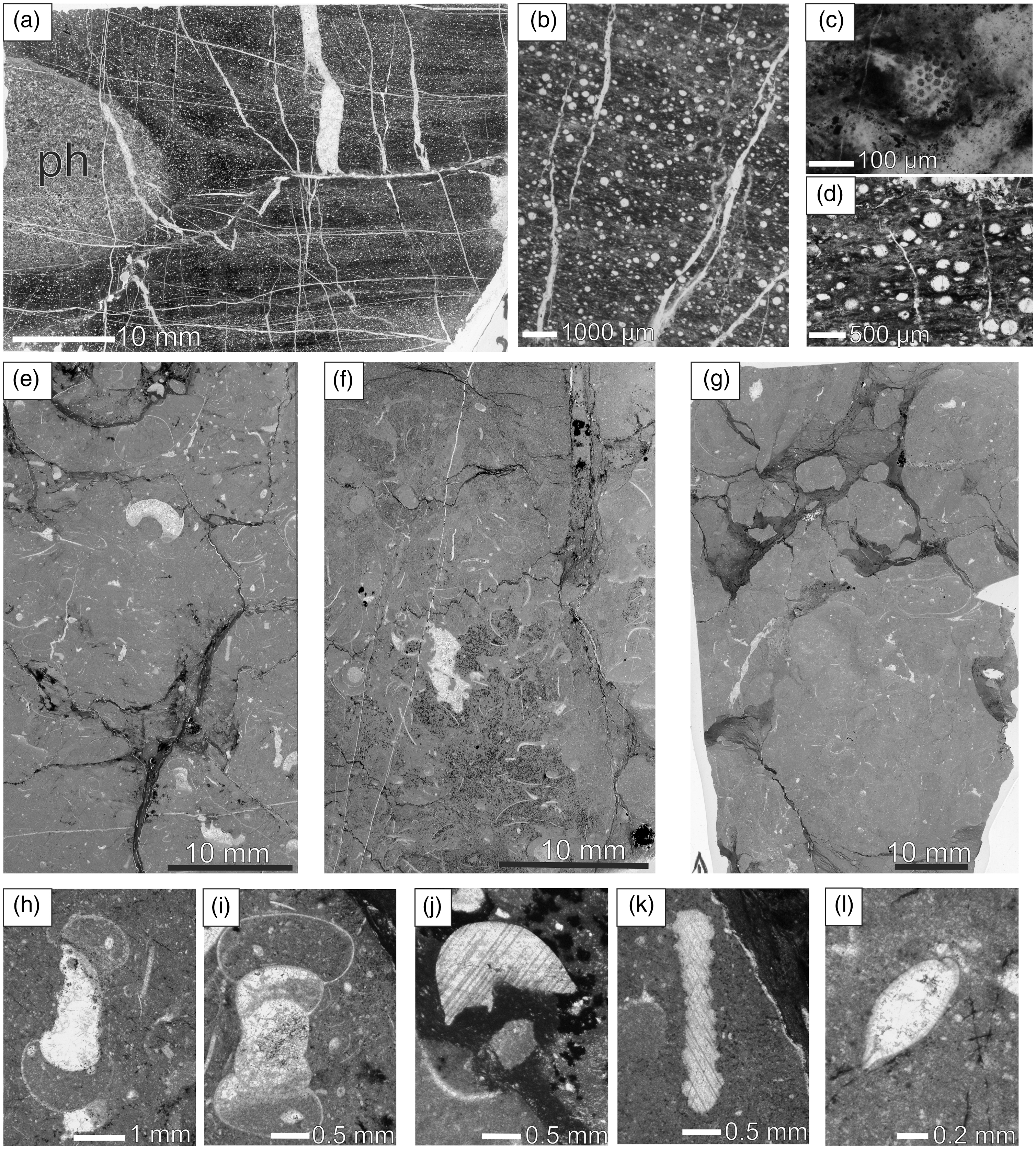
Fig. 3. (a–d) Mid-Tournaisian radiolarian cherts with numerous spumellarid radiolarians. Note the presence of phosphate nodules (ph) in (a). (e–g) Lower Tournaisian pelitic nodular limestones with numerous (e, h, i) ammonoids, (j, k) crinoid ossicles and (l) smooth-shelled ostracods.
The oldest rocks in the PDLS section involve a c. 1.5 m pale grey, micritic and marly nodular limestone with a c. 25 cm intercalation of grey shale that is an equivalent of the Hangenberg Black Shales (Fig. 2, online Supplementary Material Fig S1; Feist, Reference Feist1985; Casier et al. Reference Casier, Lethiers and Préat2001). This interval was assigned to the middle to upper praesulcata conodont Zone by Kaiser et al. (Reference Kaiser, Steuber and Becker2008), and it corresponds to the Bispathodus ultimus and Protognathodus kockeli zones (Feist et al. Reference Feist, Cornée, Corradini, Hartenfels, Aretz and Girard2021). The lower Tournaisian comprises more than 2 m of pale grey, nodular limestones that are capped by c. 70 cm of shales containing carbonate nodules (see Fig. 1c). This corresponds to the sulcata to sandbergi conodont zones (according to Kaiser et al. Reference Kaiser, Steuber and Becker2008) or sulcata to quadruplicata conodont zones according to Feist et al. (Reference Feist, Cornée, Corradini, Hartenfels, Aretz and Girard2021). The ‘mid’ Tournaisian comprises a c. 7.5 m thick unit of dark grey and black radiolarites with phosphorite nodules that are up to 6 cm in diameter, and this unit is attributed to the quadruplicata to crenulata conodont zones (e.g. Bábek et al. Reference Bábek, Kumpan, Kalvoda and Grygar2016; Feist et al. Reference Feist, Cornée, Corradini, Hartenfels, Aretz and Girard2021). The overlying Faugères Formation contains conodonts of the anchoralis conodont Zone at its base (Aretz, Reference Aretz2016).
Intervals of the PDLS section that were sampled in the present study encompass the lower Tournaisian limestones (from bed 12 sensu Kaiser et al. Reference Kaiser, Steuber and Becker2008) and ‘mid’ Tournaisian black radiolarian cherts (Fig. 1c, online Supplementary Material Figs S1, S2, S4). The studied section at Col des Tribes consists of c. 50 cm pale grey, nodular limestones that belong to the sandbergi conodont Zone (Feist et al. Reference Feist, Cornée, Corradini, Hartenfels, Aretz and Girard2021). These limestones are overlain by c. 250 cm of black and dark grey radiolarian cherts (online Supplementary Material Fig S4) which correspond to the quadruplicata to crenulata conodont zones (Bábek et al. Reference Bábek, Kumpan, Kalvoda and Grygar2016; Feist et al. Reference Feist, Cornée, Corradini, Hartenfels, Aretz and Girard2021).
Although the rocks at Montagne Noire have been thermally altered to varying degrees from 2–2.5 to 4–4.5 CAI (the conodont colour alteration index; for details see Wiederer et al. Reference Wiederer, Königschof, Feist, Franke and Doublier2002; S. I. Kaiser, unpub. Ph.D. thesis, Ruhr-Universität Bochum, 2005), the investigated rocks were not affected by the Alpine deformation. This explains the relatively good preservation of fossils and sedimentary structures in the rocks (Girard et al. Reference Girard, Cornée, Corradini, Fravalo and Feist2014), and they are thus suitable for geochemical investigations.
3. Material and methods
During fieldwork that was conducted in May 2016 the sections studied were described in detail and logs were created (see online Supplementary Material Figs S1–S4). Samples were taken bed-by-bed. A total of 43 samples were collected from the PDLS section (thickness of sampled interval was c. 10 m), whereas 20 samples were obtained from the CDT section (sampled interval was c. 3 m). The mass of the samples ranged from 500 g to 2 kg. In situ analyses of the studied sections were conducted using a GT-32 Super-Spec field-portable gamma-ray spectrometer (see online Supplementary Material Figs S5, S6). The duration of each measurement was 240 s, and this provided concentrations of potassium (K), thorium (Th) and uranium (U). The gamma-ray measurements were performed in sampled intervals, and the generated data were utilized for preliminary assessment of bottom redox conditions during sedimentation. This is because sediments deposited in bottom-water anoxic/euxinic conditions are often characterized by U enrichment (see e.g. Wignall & Myers, Reference Wignall and Myers1988; Bond & Zatoń, Reference Bond and Zatoń2003). In the PDLS section, measurements were conducted at 48 points, whereas 17 points were analysed in the CDT section. The U and Th data obtained using the portable gamma-ray spectrometry and those from inductively coupled plasma mass spectrometry (ICP-MS) were comparable (see online Supplementary Material Figs S5, S6). However, because the ICP-MS data involve higher precision values, the gamma-ray spectrometer data were treated as preliminary. In addition, 17 thin-sections for microfacies observations were made from radiolarian cherts and limestones. The thin-sections were scanned using an Epson Perfection V850 Pro scanner. Microphotographs were obtained using a Nikon Eclipse 50i transmitted light microscope with an integrated DS-Fi2 digital camera, as well as a Nikon SMZ1000 stereoscopic microscope.
The total carbon (TC), total inorganic carbon (TIC) and total sulphur (TS) contents of samples were measured using an Eltra CS-500 IR analyser that is equipped with a TIC analysis unit at the Institute of Earth Sciences, University of Silesia in Katowice, Poland. The analytical precision and accuracy were better than ±2 % for TS, ±2 % for TC and ±3 % for TIC. Total organic carbon (TOC) values were calculated as the difference between the TC and TIC (for details see e.g. Rakociński et al. Reference Rakociński, Marynowski, Zatoń and Filipiak2021 a).
Wilkin et al. (Reference Wilkin, Barnes and Brantley1996) showed that the size distribution of pyrite framboids may be a reliable proxy for redox conditions in modern marine environments. According to Wilkin et al. (Reference Wilkin, Barnes and Brantley1996), pyrite framboids form in a water column at the redox boundary in euxinic basins (lack of oxygen and presence of free H2S). Following the attainment of ≤5 μm in size, these particles rapidly sink to the bottom, and further growth is inhibited. Consequently, sediments that are deposited under euxinic conditions are enriched in pyrite framboids exhibiting narrow size distributions. Conversely, pyrite framboids that are associated with dysoxic environments (weakly oxygenated bottom waters devoid of local reactants) display larger (often >5 µm) and more variable sizes (Wilkin et al. Reference Wilkin, Barnes and Brantley1996). This method was initially tested using Jurassic sediments by Wignall & Newton (Reference Wignall and Newton1998), and subsequently, pyrite framboids have often been employed in order to decipher redox conditions prevailing in many palaeoenvironments of different ages (e.g. Zhou & Jiang, Reference Zhou and Jiang2009; Hammarlund et al. Reference Hammarlund, Dahl, Harper, Bond, Nielsen, Bjerrum, Schovsbo, Schönlaub, Zalasiewicz and Canfield2012; Huang et al. Reference Huang, Chen, Wignall and Zhao2017; Li et al. Reference Li, Song, Tian, Bond, Song, Du, Zhang, Chu, Wignall and Tong2022).
In the present study, 33 and 16 samples, respectively, from the PDLS and CDT sections were analysed using a Philips XL30 environmental scanning electron microscope (ESEM) at the Institute of Earth Sciences in Sosnowiec, Poland. Back-scattered electron imaging (BSE) was conducted on uncoated polished samples under low vacuum conditions. Regarding the size distribution analysis, we tried to measure at least 100 framboids in each sample. Full data for the framboids measured in each sample are presented in the online Supplementary Material (see online Supplementary Material Table S1; see also Figs 4, 5).
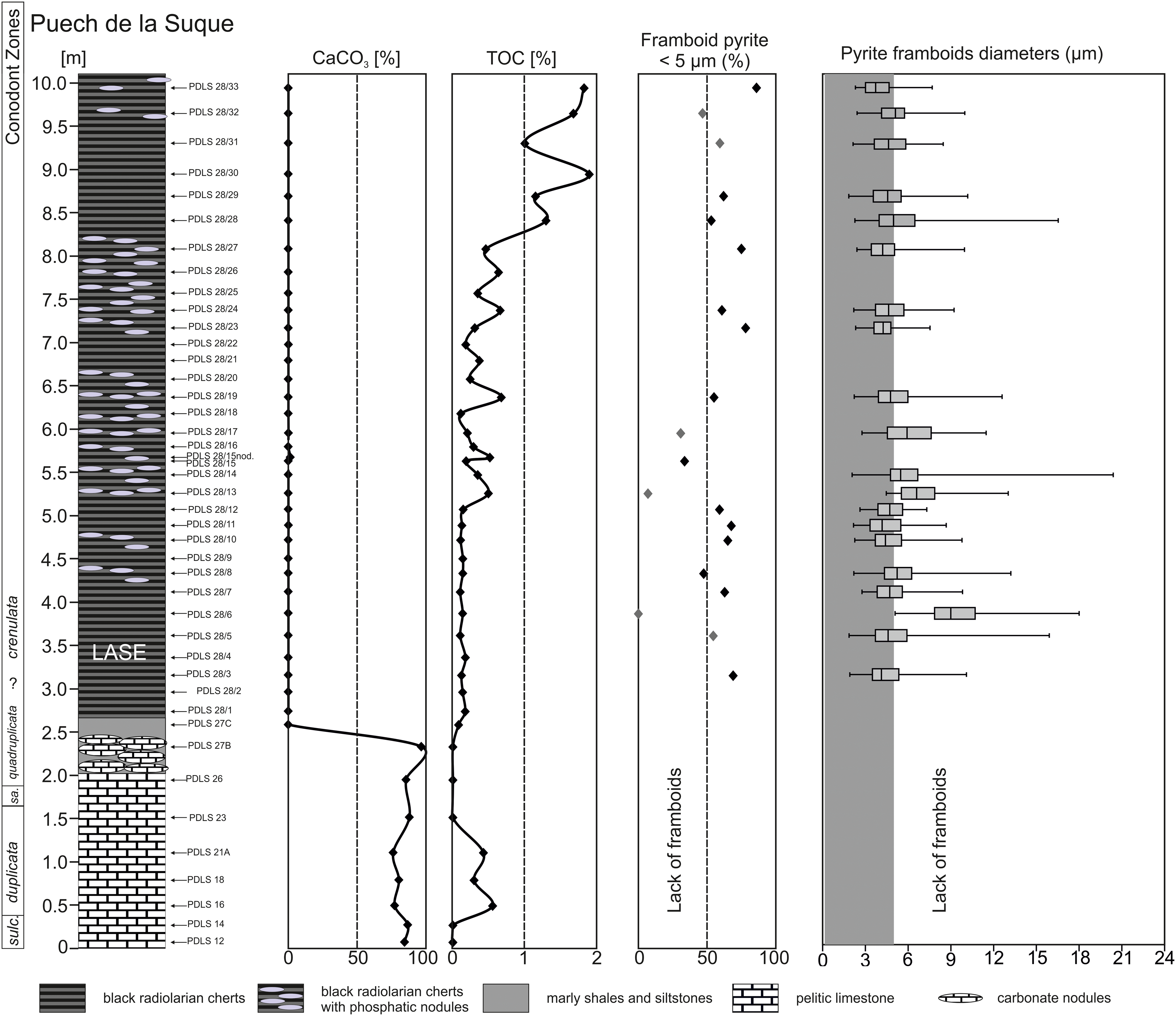
Fig. 4. Composite plot of the Devonian–Carboniferous succession at the Puech de la Suque section (Montagne Noire) showing CaCO3, total organic carbon (TOC) and framboid pyrite diameters (% <5 μm). Biostratigraphy is based on Kaiser et al. (Reference Kaiser, Steuber and Becker2008) and Feist et al. (Reference Feist, Cornée, Corradini, Hartenfels, Aretz and Girard2021).

Fig. 5. Composite plot of the Devonian–Carboniferous succession at the Col des Tribes section (Montagne Noire) showing CaCO3, total organic carbon (TOC) and framboid pyrite diameters (% <5 μm). Biostratigraphy is based on Feist et al. (Reference Feist, Cornée, Corradini, Hartenfels, Aretz and Girard2021).
Sixty-three samples were also pulverized and analysed for major, minor and trace elements using inductively coupled plasma optical emission spectrometry (ICP-OES) at the Bureau Veritas Acme Labs Canada Ltd, Canada (see online Supplementary Material Tables S2–S5). The detection was better than ±0.05 % (mostly ±0.01 %) for major elements and generally better than ±1 ppm for trace elements. Enrichment factors were used to characterize the enrichment or depletion of samples in different elements (for details see e.g. Rakociński et al. Reference Rakociński, Marynowski, Zatoń and Filipiak2021 a). Data for the radiolarian cherts and grey shales were normalized using the composition of the post-Archaean average shale (PAAS) reported by Taylor & McLennan (Reference Taylor and McLennan1985; see also Tribovillard et al. Reference Tribovillard, Algeo, Baudin and Riboulleau2012). Relatedly, data for the limestones were normalized using the average limestone composition (WED; Wedepohl, Reference Wedepohl1970). Based on experience and data from previous studies, the normalization of carbonates to the carbonate-depleted PAAS is not recommended. The composition of the average limestone standard proposed by Wedepohl (Reference Wedepohl1970) is more suitable for comparison of the elemental composition of limestones (see also Racki et al. Reference Racki, Baliński, Wrona, Małkowski, Drygant and Szaniawski2012; Pisarzowska et al. Reference Pisarzowska, Rakociński, Marynowski, Szczerba, Thoby, Paszkowski, Perri, Spalletta, Schönlaub, Kowalik and Gereke2020; Rakociński et al. Reference Rakociński, Marynowski, Zatoń and Filipiak2021 a, Reference Rakociński, Książak, Pisarzowska and Marynowski2022). Considering that some elements are clearly abundant in carbonates, a comparison of such elemental data to the average shale would unusually elevate enrichment factors (EFs) of the investigated carbonate rocks (see online Supplementary Material Tables S6, S7). The concentrations of U, for example, of the average shale (PAAS) and average limestone are correspondingly 0.31 and 2.1 ppm. Relatedly, the concentrations of P and Mn for the PAAS are 0.007 % and 0.009 %, respectively, whereas corresponding values for the average limestone are 0.03 % and 0.07 %; (see e.g. Wedepohl, Reference Wedepohl1970; Taylor & McLennan, Reference Taylor and McLennan1985). Any relative enrichment was then expressed by EF >1, and a depletion by EF <1. To decipher bottom redox conditions in the deep pelagic settings of the Montagne Noire region, the U/Th, V/Cr and Corg/P ratios and concentrations of redox-sensitive elements (U, Mo, V, Ni, Pb, Cu and Co) (e.g. Tribovillard et al. Reference Tribovillard, Algeo, Lyons and Riboulleau2006; Algeo & Ingall, Reference Algeo and Ingall2007; Rakociński et al. Reference Rakociński, Marynowski, Zatoń and Filipiak2021 a), as well as the diameters of pyrite framboids (e.g. Wignall & Newton, Reference Wignall and Newton1998; Bond & Wignall, Reference Bond and Wignall2010), were utilized.
The concentrations of mercury (Hg) in the samples were analysed using a dual-cell pyrolyser-type Milestone DMA-80 Direct Mercury Analyser for atomic absorption spectrometry at the Institute of Earth Sciences, University of Silesia in Katowice, Poland. The detection limit was 0.2 ppb, and analytical details of the analysis reported in Racki et al. (Reference Racki, Marynowski and Rakociński2018 a).
4. Results
4.a. Microfacies, total organic carbon, total sulphur and carbonate contents
The lower Tournaisian nodular and pelitic limestones are developed as wackestone with numerous goniatites. The fossil assemblage also includes smooth-shelled ostracods, shell debris, crinoid ossicles and fragments of trilobites (Fig. 3). The micritic, matrix-supported wackestones are bioturbated and exhibit microstylolites. Radiolarians are present in the uppermost part of these limestones. The mid-Tournaisian black siliceous rocks investigated, referred to as bedded cherts in older reports (e.g. Aretz, Reference Aretz2016), are developed as black and dark grey radiolarites containing abundant spumellarian radiolarians and siliceous mud with phosphatic concretions occurring locally (Fig. 3).
Total organic carbon and carbonate contents in the PDLS and CDT sections are variable. The CaCO3 contents of limestone samples that were obtained from the CDT and PDLS sections range correspondingly from 80.47 to 90.84 wt % and from 76.24 to 96.67 wt % (Tables 1, 2; Figs 4, 5). In contrast, chert samples from both sections yielded no or trace amounts of CaCO3 (Tables 1, 3; Figs 4, 5), excluding a phosphatic nodule (sample PDLS 15nod), which yielded CaCO3 reaching 1.45 wt % (Table 1). Limestones in both sections are poor in organic matter, the TOC ranging from 0.01 to 0.12 wt % in the CDT section (Table 2; Fig. 5), and from 0.01 to 0.56 wt % in the PDLS (Table 1; Fig. 4). In the black radiolarian cherts, TOC contents differ between investigated sections. In the CDT section the TOC contents range from 0.40 to 1.36 wt % (generally above 0.5 wt %; Table 2). Conversely, those of samples from the PDLS section (0.09–1.90 wt %) are mostly <0.2 wt %, but increase from the lower to the upper portion of the section (Table 1; Fig. 4). Total sulphur is relatively low across the whole succession in both sections (generally reaching zero or trace amounts; see Tables 1, 2).
Table 1. Geochemical data from the lower (limestone) and middle Tournaisian (black radiolarian cherts) at the Puech de la Suque section: TOC, TS, CaCO3 and Hg contents and U/Th, V/Cr, Corg/P, Corg/P(molar), Hg/TOC, Hg/Al and Hg/Mo ratios

* Non-reliable values of Hg/TOC ratio (<0.2 wt % TOC).
Table 2. Geochemical data from the lower (limestone) and middle Tournaisian (black radiolarian cherts) at the Col de Tribes section: TOC, TS, CaCO3 and Hg contents and U/Th, V/Cr, Corg/P, Corg/P(molar), Hg/TOC, Hg/Al and Hg/Mo ratios

* Non-reliable values of Hg/TOC ratio (<0.2 wt % TOC).
Table 3. Enrichment factors of selected major and trace elements in the Puech de la Suque section

Abbreviation: avg. EF – average enrichment factor. Sample 28/15nod# – phosphatic-carbonate nodule normalized to average limestone of Wedepohl (Reference Wedepohl1970), excluded from calculation of avg. EF in black radiolarian cherts.
4.b. Pyrite framboids
Among 33 samples that were obtained from the PDLS section, 11 were devoid of pyrite framboids. Regarding the other 22 samples, 15 enabled the measurement of at least 100 framboids, whereas seven allowed for measurements of at least 30 specimens. Relatedly, among 16 samples that were collected from the CDT section, nine samples contained no pyrite framboids, whereas at least 100 framboids were measured in one sample. In the other six samples, 23 to 61 framboids were measured. The samples devoid of pyrite framboids contained other forms of pyrite (microconcretions, euhedral crystals).
The sizes of framboids for samples that were collected from the PDLS section range from 1.9 to 20.4 μm, with a mean of 3.9 to 9.7 μm, and are characterized by low standard deviations (0.9–2.9). Among 14 samples, >50 % of the measured framboids yielded a diameter of <5 μm (Fig. 4, online Supplementary Material Table S1). Regarding samples that were obtained from the CDT section, the sizes of framboids range from 2.0 to 17.9 μm (mean ranging from 4.4 to 7.0 μm) and also produced low standard deviations (1.2–2.5). In three samples, >50 % of measured framboids show diameters of <5 μm (Fig. 5, online Supplementary Material Table S1).
4.c. Inorganic geochemistry data and Corg/P ratios
Phosphorus in the limestones is depleted with avg. P(EF) = 0.26 in the PDLS and avg. P(EF) = 0.49 in the CDT. The black radiolarian cherts exhibit widely variable P(EF) values that range from 1.06 to 419.14 (but generally high with avg. P(EF) = 87.57; Table 4) for samples that were collected from the CDT and 0.69 to 840.38 (but generally high with avg. P(EF) = 59.46; Table 3) for samples that were obtained from the PDLS section.
Table 4. Enrichment factors of selected major and trace elements in the Col de Tribes section

Abbreviation: avg. EF – average enrichment factor.
The weight Corg/P ratio values in the PDLS are relatively low ranging from 7.64 to 42.81 for the limestones (generally <30; Table 1; Fig. 6) and from 0.06 to 52.04 for the black radiolarian cherts (mostly <30; Table 1; Fig. 6). The lowest value of 0.04 was obtained from a phosphatic nodule sample (sample PDLS 28/15 nod; Table 1). In the CDT section, the Corg/P ratios are also low, ranging from 4.58 to 4.76 in the limestones and from 0.15 to 61.75 in the radiolarian cherts (mainly below 30; Table 2; Fig. 7).
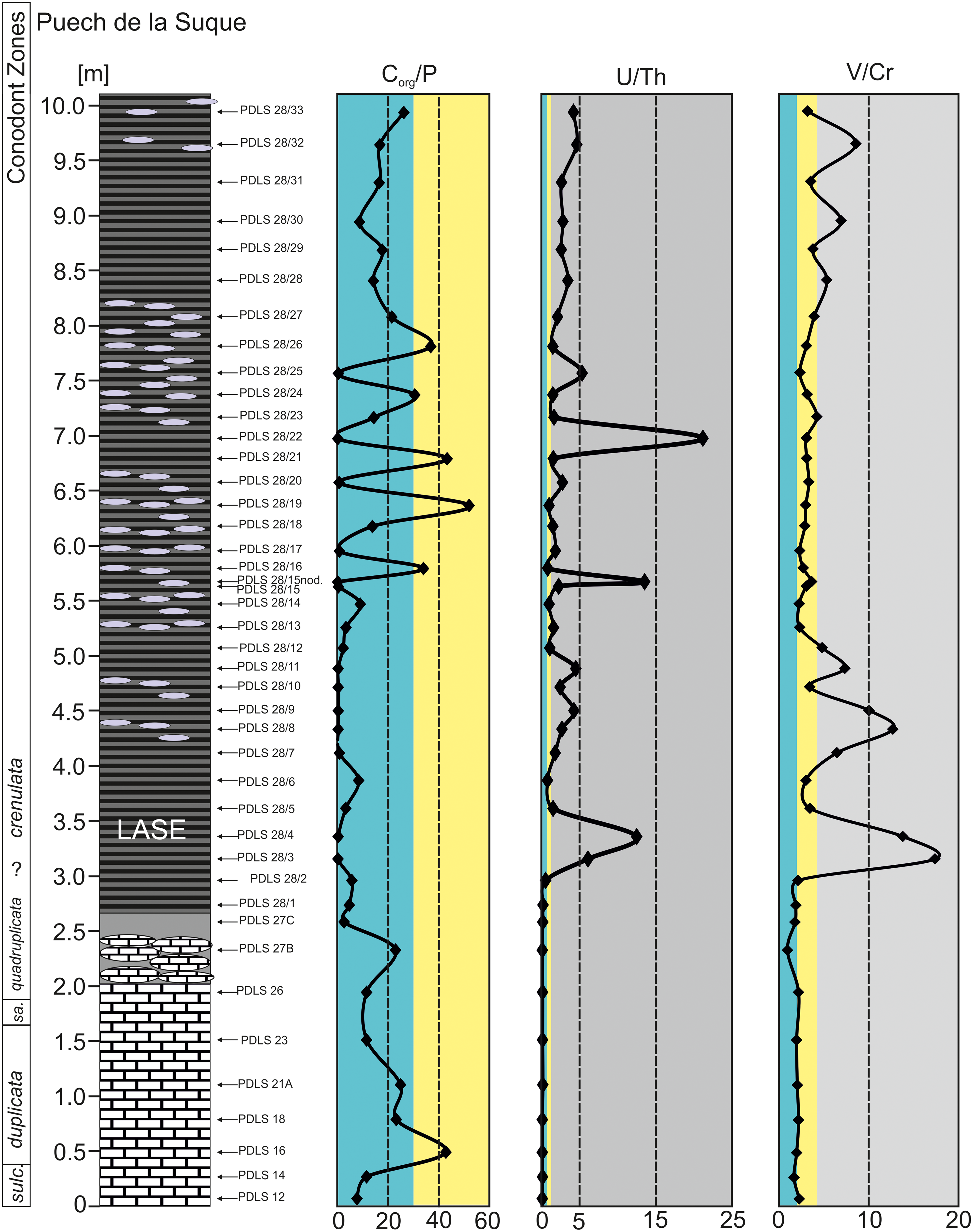
Fig. 6. Composite plot of the Tournaisian succession at the Puech de la Suque section showing Corg/P, U/Th and V/Cr ratios. For an explanation of lithology and abbreviations see Figure 4. Blue – oxic conditions; yellow – dysoxic conditions; grey – anoxic conditions.

Fig. 7. Composite plot of the Tournaisian succession at the Col des Tribes section showing Corg/P, U/Th and V/Cr ratios. For an explanation of lithology and abbreviations see Figure 5. Blue – oxic conditions; yellow – dysoxic conditions; grey – anoxic conditions.
The uranium contents in the limestones are low in both sections, below 0.6 ppm in the PDLS (with avg. U(EF) = 0.11; Fig. 8; Table 3, online Supplementary Material Table S4) and below 0.9 ppm in the CDT (with avg. U(EF) = 0.22, Fig. 9; Table 4, online Supplementary Material Table S5). Evidently, the black radiolarian cherts exhibit higher U(EF) values with avg. U(EF) = 11.92 in the PDLS and avg. U(EF) = 10.42 in the CDT (see Figs 8, 9; Tables 3, 4). However, the grey shales and lowermost radiolarian cherts in the PDLS section are depleted in uranium (Table 3). The U/Th ratios in limestones from both sections are low (<0.3). Values of U/Th in the radiolarian cherts and shale in the PDLS section are variable, ranging from 0.18 to 21.20 (generally >1.25; Table 1; Fig. 6), while in the CDT section, the U/Th ratios range from 1.57 to 6.68 (Table 2; Fig. 7). The V/Cr ratios of limestone samples that were collected from the PDLS section range from 0.95 to 2.27 (Table 1; Fig. 6) and from 1.61 to 2.78 in the CDT section (Table 2; Fig. 7). In the black radiolarian cherts, V/Cr values generally increase, ranging from 1.80 to 17.39 (mainly >2) in the PDLS section (Table 1; Fig. 6) and from 0.90 to 16.03 (mostly >3) for the CDT section (Table 2; Fig. 7).

Fig. 8. Composite plot of the Tournaisian succession at the Puech de la Suque section showing Al2O3 (%) contents and enrichment factors of Mo(EF), U(EF) and V(EF). For an explanation of lithology and abbreviations see Figure 4.

Fig. 9. Composite plot of the Tournaisian succession at the Col des Tribes section showing Al2O3 (%) contents and enrichment factors of Mo(EF), U(EF) and V(EF). For an explanation of lithology and abbreviations see Figure 5.
The concentrations of Mo in the limestone samples that were collected from both sections range from 0.3 to 2.1 ppm, whereas values for the black radiolarian cherts vary from 0.4 to 21.0 ppm for the PDLS (online Supplmentary Material Tables S4–S5) and from 0.4 to 5.8 ppm for the CDT sections. The limestones are generally depleted or slightly enriched in Mo (with avg. Mo(EF) = 1.54 at PDLS and avg. Mo(EF) = 1.33 at CDT, respectively; Figs 8, 9; Tables 3, 4). Conversely, for samples that were collected from the black radiolarian cherts, Mo(EF) reflects higher enrichment, ranging from 5.18 to 66.44 (with avg. Mo(EF) = 19.38 at CDT; Fig. 9; Table 4), while in the PDLS section it ranges from 1.75 to 330.75 (with avg. Mo(EF) = 26.68; Fig. 8; Table 3).
Limestone samples that were collected from the CDT section produced V and Ni concentrations that are close to average limestones with avg. V(EF) = 1.02 and Ni(EF) = 1.26 in the CDT (Figs 8, 9; Table 4). In the PDLS section, V values are closely similar to average limestones with avg. V(EF) = 0.97, while Ni contents slightly increase, reaching avg. Ni(EF) = 1.64 (Table 3). The black radiolarian cherts are more vanadium enriched in comparison to the PAAS, with avg. V(EF) = 10.40 in the PDLS and V(EF) = 8.98 in the CDT (Figs 8, 9; Tables 3, 4). Ni is enriched in the PDLS with avg. Ni(EF) = 4.66, and in the CDT with avg. Ni(EF) = 4.74 (Tables 3, 4). Pb is depleted in the limestones with avg. Pb(EF) = 0.54 in the PDLS and Pb(EF) = 0.59 in the CDT, while in the black radiolarian cherts, EFs increase, with Pb(EF) = 3.64 in the CDT and Pb(EF) = 4.38 in the PDLS (Tables 3, 4). Copper is enriched in both sections in the limestones, as well as in the black radiolarian cherts (3.44 to 4.04 in the limestones and 9.89 to 11.12 avg. Cu(EF) in the cherts; Tables 3, 4). Regarding Zr, contents show values from 7.0 to 124.5 ppm at the PDLS section and from 10.8 to 45.6 ppm at the CDT section (online Supplementery Material Tables S4–S5). The samples that were obtained from the black radiolarian cherts are depleted in Zr relative to the PAAS (with avg. Zr(EF) = 0.86 in both sections; Tables 3, 4). Conversely, in the limestone, Zr contents are close to the average limestone compositions (with avg. Zr(EF) = 1.01 in the PDLS and avg. Zr(EF) = 1.20 in the CDT; see Tables 3, 4).
4.d. Mercury contents
Mercury contents in the limestones are low, ranging from 5.52 to 22.53 ppb in the PDLS section and 17.62 to 28.30 ppb in the CDT section (Figs 10, 11; Tables 1, 2). In the black radiolarian cherts, mercury contents increased, ranging from 48.29 to 222.99 ppb in the CDT and from 59.26 to 883.48 ppb in the PDLS (in both sections most samples have Hg >100 ppb; Tables 1, 2). In the PDLS, three mercury spikes above 500 ppb are observed (Fig. 10; Table 1). The first Hg spike occurs in the silica-rich interval that is an equivalent of the LASE (Fig. 10). However, the Hg/TOC ratio values are unreliable for samples with low TOC contents (<0.2 wt %; see Sial et al. Reference Sial, Chen, Lacerda, Frei, Tewari, Pandit, Gaucher, Ferreira, Cirilli, Peralta, Korte, Barbosa and Pereira2016; Percival et al. Reference Percival, Ruhl, Hesselbo, Jenkyns, Mather and Whiteside2017; Grasby et al. Reference Grasby, Them, Chen, Yin and Ardakani2019). Therefore, this ratio is inapplicable to the lower part of the PDLS section that is characterized by low TOC values (Table 1; Fig. 10). However, in the upper part of the cherts in the intervals with Hg spikes, the Hg/TOC ratio and the TOC contents increase, which could confirm the volcanic source of Hg (e.g. Grasby et al. Reference Grasby, Them, Chen, Yin and Ardakani2019). The Hg/TOC ratio for limestones that were collected from the CDT section are unreliable, whereas values for samples that were obtained from the black radiolarian cherts range from 83.71 to 514.56 ppb/%, and this ratio increases as the concentration of Hg in the samples increases (Table 2). The Hg/TS ratio is generally very high in both sections, which is associated with the strong depletion of TS (Tables 1, 2). The Hg/Al ratio is lower in the limestones, ranging from 3.42 to 56.03 ppb/% (Tables 1, 2), while in the black radiolarian cherts it increases and ranges from 10.65 to 1015.13 in the PDLS (Table 1) and from 62.50 to 162.69 ppb/% in the CDT (Table 2). The Hg/Al spikes are very well correlated with Hg spikes (Figs 10, 11). The Hg versus TOC correlations in the investigated sections were very low (R2 = 0.28, p < 0.05 in the PDLS and R2 = 0.15, p > 0.05 in the CDT), the Hg versus TS correlations were relatively low (R2 = 0.47, p < 0.05 in the PDLS and R2 = 0.15, p > 0.05 in the CDT), whereas the Hg versus Mo correlations were also weak to moderate (R2 = 0.15 in the CDT and R2 = 0.67 in the PDLS; all p < 0.05), indicating no or weak correlations with redox changes. The Hg versus Al2O3 reflected no correlations (R2 = 0.0002 in the PDLS and R2 = 0.001 in the CDT; all p > 0.05), indicating no correlation with clay fractions and terrigenous input. The impoverished TS in both sections (Tables 1, 2) excludes the sulphide fraction as a host of mercury. Generally, low correlation or lack of correlation of Hg with the above elements is indicative of a volcanic Hg source (e.g. Sial et al. Reference Sial, Chen, Lacerda, Frei, Tewari, Pandit, Gaucher, Ferreira, Cirilli, Peralta, Korte, Barbosa and Pereira2016; Racki, Reference Racki2020; Rakociński et al. Reference Rakociński, Pisarzowska, Corradini, Narkiewicz, Dubicka and Abdiyev2021 b, Reference Rakociński, Książak, Pisarzowska and Marynowski2022).
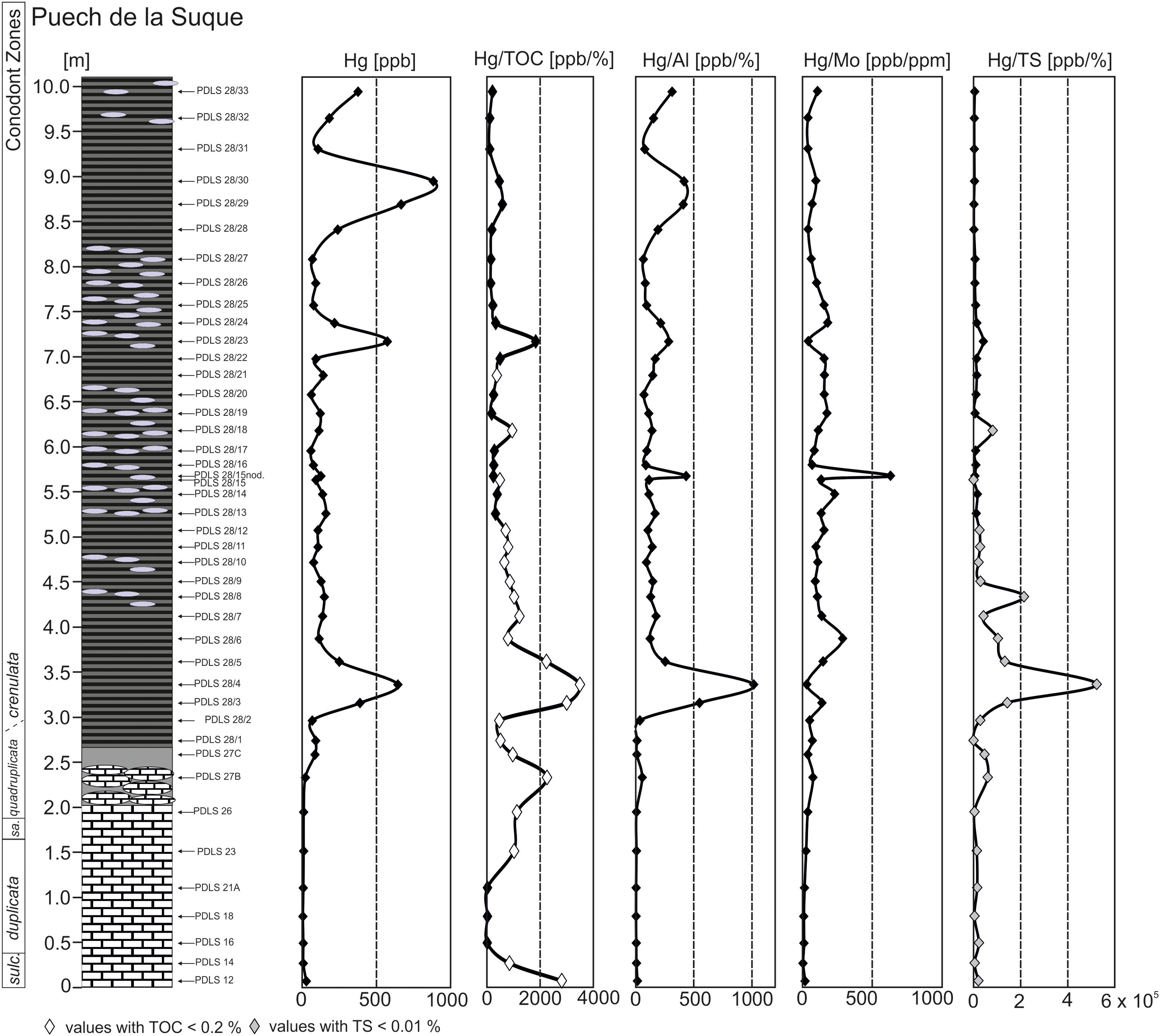
Fig. 10. Composite plot of the Tournaisian succession at the Puech de la Suque section showing Hg (ppb) contents and Hg/TOC (ppb/%), Hg/Al (ppb/%), Hg/Mo (ppb/ppm) and Hg/TS (ppb/%) ratio contents (values with TOC <0.2 are marked in white; TS <0.01 % are marked in grey). For an explanation of lithology and abbreviations see Figure 4.

Fig. 11. Composite plot of the Tournaisian succession at the Col de Tribes section showing Hg (ppb) contents and Hg/TOC (ppb/%), Hg/Al (ppb/%) and Hg/Mo (ppb/ppm) ratios (values with TOC <0.2 are marked in white). For an explanation of lithology and abbreviations see Figure 5.
5. Discussion
5.a. Conditions during deposition of the lower Tournaisian limestones
The low values of Corg/P (<30) in both sections (with the exception of sample PDLS 16 reaching 42.81; Table 1) are typical of oxic conditions (Algeo & Ingall, Reference Algeo and Ingall2007; for details see Fig. 12). Similarly, the low U/Th (<0.75) values in the limestones in both sections are indicative of oxic conditions (Jones & Manning, Reference Jones and Manning1994; Marynowski et al. Reference Marynowski, Zatoń, Rakociński, Filipiak, Kurkiewicz and Pearce2012; Rakociński et al. Reference Rakociński, Zatoń, Marynowski, Gedl and Lehman2018, Reference Rakociński, Marynowski, Zatoń and Filipiak2021 a). In contrast, the V/Cr ratios reaching values of <2 are typical of oxic conditions, and those between 2 and 4.25 indicate dysoxic conditions (see Fig. 12) during deposition of the limestones (Figs 6, 7; Tables 1, 2). However, according to Algeo & Liu (Reference Algeo and Liu2020), caution is required when a fixed metal redox proxy, such as V/Cr (used as a provenance indicator; Bock et al. Reference Bock, McLennan and Hanson1998), is applied. In fact, the U/Th ratio and enrichments of redox-sensitive trace metals are more reliable indicators of redox conditions than the V/Cr ratio (e.g. Rakociński et al. Reference Rakociński, Pisarzowska, Janiszewska and Szrek2016, Reference Rakociński, Marynowski, Zatoń and Filipiak2021 a; Algeo & Liu, Reference Algeo and Liu2020).

Fig. 12. Schematic drawing showing the relationship between faunal diversity bioturbation degree, pyrite framboid occurrence, pyrite taphofacies, inorganic geochemistry and the environmental conditions at the sea-floor (after Brett et al. Reference Brett, Dick, Baird, Landing and Brett1991; Wignall, Reference Wignall1993; Jones & Manning, Reference Jones and Manning1994; Allison et al. Reference Allison, Wignall, Brett, Bosence and Allison1995; Racki et al. Reference Racki, Piechota, Bond and Wignall2004; Algeo & Ingall, Reference Algeo and Ingall2007).
The limestones are depleted in redox-sensitive elements such as U, Zn and Pb (with avg. U(EF) = 0.11 in the PDLS and avg. U(EF) = 0.22 in the CDT; avg. Zn(EF) = 0.26 in the PDLS and avg. Zn(EF) = 0.52 in the CDT; avg. Pb(EF) = 0.54 in the PDLS and avg. Pb(EF) = 0.59 in the CDT; Tables 3, 4). Vanadium reached values close to average limestones (avg. V(EF) = 0.97 in the PDLS and avg. V(EF) = 1.02 in the CDT). Other elements such as Mo and Ni are slightly enriched in some samples in both sections (Tables 3, 4). However, most of the samples reflected depletion in Mo, Ni and V. All of these proxies suggest that during sedimentation of the lower Tournaisian ‘Griotte’ limestones in the Montagne Noire, well-oxygenated bottom-water conditions prevailed (Fig. 13b). It is also confirmed by the lack of pyrite framboids in all samples studied.

Fig. 13. Depositional model of sedimentation and palaeoenvironmental conditions in the Montagne Noire during early Carboniferous time. (a) The mid-Tournaisian part of the succession, an equivalent of the LASE (Lydiennes Fm), showing periodical anoxic to dysoxic bottom-water conditions, and episodic anoxia in the water column. (b) The lower Tournaisian part of the succession (‘supragriotte’ limestones) was formed in oxic or weakly dysoxic bottom-water conditions.
5.b. Conditions during deposition of the mid-Tournaisian black radiolarian cherts
The U/Th ratios in the black radiolarian cherts from both sections are generally high (>1.25), also pointing to anoxic bottom-water conditions during their deposition (Figs 6, 7; Tables 1, 2); only values in a few samples from the PDLS section, ranging between 0.75 and 1.25, are indicative of dysoxic conditions (e.g. Jones & Manning, Reference Jones and Manning1994; Racka et al. Reference Racka, Marynowski, Filipiak, Sobstel, Pisarzowska and Bond2010; Rakociński et al. Reference Rakociński, Marynowski, Zatoń and Filipiak2021 a, Reference Rakociński, Książak, Pisarzowska and Marynowski2022; see also Fig. 12). The V/Cr ratio values are variable. In the lowermost samples from the PDLS section they reach a value below 2, indicating oxic conditions, and between 2 and 4.25, typical of dysoxic conditions (Jones & Manning, Reference Jones and Manning1994; Racka et al. Reference Racka, Marynowski, Filipiak, Sobstel, Pisarzowska and Bond2010; Mouro et al. Reference Mouro, Rakociński, Marynowski, Pisarzowska, Musabelliu, Zatoń, Carvalho, Fernandes and Waichel2017; Rakociński et al. Reference Rakociński, Marynowski, Zatoń and Filipiak2021 a, Reference Rakociński, Książak, Pisarzowska and Marynowski2022; see also Fig. 12). The V/Cr ratios, which are generally >4.25 for samples from the lower part of the black radiolarian cherts, are characteristic of anoxic conditions (Fig. 6; Table 1). In the middle and upper part of the succession, the V/Cr values are typical of dysoxic conditions (Fig. 6; Table 1). In the CDT section, the V/Cr values are indicative for anoxic–dysoxic conditions, with the exception of one sample with low V/Cr values <2, typical of oxic conditions (Fig. 7; Table 2; e.g. Mouro et al. Reference Mouro, Rakociński, Marynowski, Pisarzowska, Musabelliu, Zatoń, Carvalho, Fernandes and Waichel2017; Rakociński et al. Reference Rakociński, Marynowski, Zatoń and Filipiak2021 a, Reference Rakociński, Książak, Pisarzowska and Marynowski2022). The black radiolarian cherts generally exhibit enrichments in redox-sensitive trace elements, such as U, Mo, V, Ni, Pb, Cu and Co (Tables 3, 4), which indicate anoxic bottom-water conditions during deposition of these sediments (e.g. Tribovillard et al. Reference Tribovillard, Algeo, Lyons and Riboulleau2006; Calvert & Pedersen, Reference Calvert and Pedersen2007; Bennett & Canfield, Reference Bennett and Canfield2020).
Phosphorous in the investigated black radiolarian cherts reflected high enrichments with avg. P(EF) = 59.46 in the PDLS section, and P(EF) = 87.57 in the CDT section; however, the P(EF) values are variable between particular samples (Tables 3, 4). The Corg/P values in both sections are relatively low and generally below 30 (Figs 6, 7; Tables 1, 2), and these values are indicative of oxic conditions. A few samples show values between 30 and 150 (generally <60), and these are typical of dysoxic or periodic anoxic conditions (e.g. Algeo & Ingall, Reference Algeo and Ingall2007; Pisarzowska et al. Reference Pisarzowska, Rakociński, Marynowski, Szczerba, Thoby, Paszkowski, Perri, Spalletta, Schönlaub, Kowalik and Gereke2020; Rakociński et al. Reference Rakociński, Marynowski, Zatoń and Filipiak2021 a). The variability associated with the Corg/P data reflect changes in the intensity of the bottom-water anoxia. The molar Corg/P ratios (applied by Algeo & Ingall, Reference Algeo and Ingall2007) reached higher values (Tables 1, 2); however, generally it is indicative of oxic–dysoxic or periodically anoxic conditions with the exception of single samples in the CDT section (Table 2).
Fluctuations between dysoxic and anoxic conditions are also reflected by different size-frequency distributions of pyrite framboids of the samples (Figs 4, 5, online Supplementary Material Table S1). Apart from the samples devoid of framboids there are assemblages dominated by small-sized specimens (<5 μm, low standard deviations) typical of anoxic conditions, and populations dominated by framboids >5 μm, where larger forms (>10 μm, and higher standard deviations) also occur, indicating a better-oxygenated palaeoenvironment (e.g. Bond & Wignall, Reference Bond and Wignall2010; Rakociński et al. Reference Rakociński, Zatoń, Marynowski, Gedl and Lehman2018). Generally, a high concentration of phosphorous and numerous phosphatic concretions in the whole mid-Tournaisian part of the successions consisting of black radiolarian cherts, in connection with not very high TOC contents (Figs 4, 5), which even in the LASE interval do not exceed 2 %, are indicative of non-sulphidic conditions (cf. März et al. Reference März, Poulton, Beckmann, Küster, Wagner and Kasten2008).
The elevated concentrations of U, Mo, V, Cu and Pb observed in the lower part of the black radiolarian cherts (c. 50–80 cm above the base, samples 28/3–28/4; Fig. 8) in the PDLS section correspond to those from the lower part of the black radiolarian cherts succession at the CDT section (Fig. 9). In the upper part of the PDLS section, another level of elevated U and Mo contents is observed (Fig. 8; Table 1; in sample 28/22). These intervals may reflect more oxygen-restricted conditions.
Generally, during the deposition of the mid-Tournaisian Lydiennes Formation in the Montagne Noire region, dysoxic to anoxic (but non-sulphidic) bottom-water conditions prevailed (Fig. 13). Our results demonstrate that, on a regional scale, the mid-Tournaisian anoxic event (LASE) in pelagic settings was not uniform. The data obtained from the Montagne Noire successions that were investigated do not reflect strong anoxic conditions, such as those reported for the Polish part of the southern Laurussian shelf (Rakociński et al. Reference Rakociński, Marynowski, Zatoń and Filipiak2021 a) and the Carnic Alps (Rakociński et al. Reference Rakociński, Książak, Pisarzowska and Marynowski2022).
These results closely correspond with those of Borrego et al. (Reference Borrego, López García and Merino-Tomé2018), who investigated the mid-Tournaisian successions in the Cantabrian Zone in Spain. The authors also documented such variable conditions in the LASE-equivalent ‘mid’ to upper Tournaisian organic-rich black shales and radiolarites (Vegamián Formation). The investigated sediments of the Vegamián Formation are characterized by higher TOC values, ranging from 2.8 to 7.4 wt .%, than in the Montagne Noire. Gamma-ray spectrometry and biomarker data, however, suggested an oxic to dysoxic depositional environment with only local anoxic conditions detected at the base of the Vegamián Formation (Borrego et al. Reference Borrego, López García and Merino-Tomé2018). This is in agreement with the conclusions of Bábek et al. (Reference Bábek, Kumpan, Kalvoda and Grygar2016), who previously investigated several samples of mid-Tournaisian sediments at e.g. Montagne Noire (Puech de la Suque), the Carnic Alps (Kronhofgraben) and Rhenish Massif (Drewer) and stated that the LASE sediments were formed in dysoxic/anoxic conditions with elevated pelagic production of siliceous skeletons (see fig. 11 in Bábek et al. Reference Bábek, Kumpan, Kalvoda and Grygar2016). These data also generally correspond with the conclusions of Siegmund et al. (Reference Siegmund, Trappe and Oschmann2002), who described the LASE in the Rhenish Massif, Germany. However, according to Siegmund et al. (Reference Siegmund, Trappe and Oschmann2002), LASE sediments in the German part of Laurussia were formed in eutrophic and generally anoxic or even euxinic conditions, which was confirmed by e.g. high values of V/Cr ratios (>4.25).
Results obtained from the Polish part of the Laurussian shelf (Rakociński et al. Reference Rakociński, Marynowski, Zatoń and Filipiak2021 a), Carnic Alps (Rakociński et al. Reference Rakociński, Książak, Pisarzowska and Marynowski2022) and Montagne Noire (this study) confirm that LASE sediments were deposited under variable bottom-water conditions. The depositional environment did not always witness strong anoxic/euxinic conditions as in the case of the Polish and Austrian successions. The relatively lower values of Corg/P ratios and TOC contents in the Montagne Noire during the mid-Tournaisian seemingly do not confirm elevated phytoplankton productivity in the photic zone during the LASE. Both investigated sections reflected moderate, not exceeding 2 %, TOC contents even in the LASE interval. However, potentially higher primary organic matter contents could have been degraded during episodes of bottom-water oxygenation in environments that were characterized by low sedimentation rates (e.g. Canfield, Reference Canfield1994). Slow sedimentation rates and condensation were postulated during the LASE by e.g. Siegmund et al. (Reference Siegmund, Trappe and Oschmann2002) and Borrego et al. (Reference Borrego, López García and Merino-Tomé2018). It can be confirmed by generally low sedimentation rates less than 1 m/Ma during Tournaisian time in the Montagne Noire (Aretz, Reference Aretz2016). On the other hand, during the LASE and deposition of the younger part of the Lydiennes Formation, elevated productivity occurred. This is marked by the radiolarian bloom observed in thin-sections of the black radiolarian cherts from the PDLS and CDT sections (see Fig. 3; see also Bábek et al. Reference Bábek, Kumpan, Kalvoda and Grygar2016). In fact, these two processes can overlap.
Sedimentary rocks in the Montagne Noire have low TOC and TS contents, which could be interpreted as the effect of weathering or palaeoweathering (see e.g. Wildman et al. Reference Wildman, Berner, Petsch, Bolton, Eckert, Mok and Evans2004; Marynowski et al. Reference Marynowski, Kurkiewicz, Rakociński and Simoneit2011). However, an intense diagenetic overprint can be excluded because many samples contain numerous pyrite framboids. Their numbers should decrease or even totally vanish in the weathered zone (Marynowski et al. Reference Marynowski, Kurkiewicz, Rakociński and Simoneit2011). In addition, the rocks investigated are enriched in Mo and U, while in the weathered sediments, contents of these elements decrease (see Marynowski et al. Reference Marynowski, Pisarzowska, Rakociński, Derkowski, Środoń, Szaniawski and Cohen2017).
Despite the traditional interpretation that most of the organic-rich sediments were formed under anoxic/euxinic conditions, they can be formed in variable sedimentary regimes. For example, Rimmer (Reference Rimmer2004) and Rimmer et al. (Reference Rimmer, Thompson, Goodnight and Robl2004) documented variable bottom-water conditions during sedimentation of Upper Devonian and Mississippian organic-rich shales from the Central Appalachian Basin (USA). Schieber (Reference Schieber2009), based on the occurrence of agglutinated foraminifera in Upper Devonian black shales of the Appalachian and Illinois basins, also documented different sedimentary conditions, not only euxinic bottom waters. Different oxygenation regimes and/or short-term oxygenation were also detected during other anoxic events in Late Devonian time such as e.g. the Lower Kellwasser Event in the Holy Cross Mountains in Poland (Rakociński et al. Reference Rakociński, Pisarzowska, Janiszewska and Szrek2016) and Montagne Noire (Bond et al. Reference Bond, Wignall and Racki2004). Short-term oxygenation of bottom waters under generally euxinic conditions has been reported during Famennian anoxic events, such as the Annulata event (Racka et al. Reference Racka, Marynowski, Filipiak, Sobstel, Pisarzowska and Bond2010), Dasberg event (Marynowski et al. Reference Marynowski, Filipiak and Zatoń2010) and Hangenberg Black Shale Event (Kazmierczak et al. Reference Kazmierczak, Kremer and Racki2012; Marynowski et al. Reference Marynowski, Zatoń, Rakociński, Filipiak, Kurkiewicz and Pearce2012) in the Holy Cross Mountains. Such conditions have also been associated with the Hangenberg Black Shale Event in the Carnic Alps and Rhenish Massif (Pisarzowska et al. Reference Pisarzowska, Rakociński, Marynowski, Szczerba, Thoby, Paszkowski, Perri, Spalletta, Schönlaub, Kowalik and Gereke2020) and deposition of the Cleveland Shale of the Appalachian Basin in the USA (Martinez et al. Reference Martinez, Boyer, Droser, Barrie and Love2019). Rakociński et al. (Reference Rakociński, Zatoń, Marynowski, Gedl and Lehman2018) investigated uppermost Jurassic and Lower Cretaceous organic-rich siltstones from Spitsbergen, which were also deposited under a high-productivity regime with oxic/dysoxic bottom-water conditions. Based on a high-resolution geochemical investigation of the Coniacian–Santonian Oceanic Anoxic Event 3, März et al. (Reference März, Poulton, Beckmann, Küster, Wagner and Kasten2008) stated that during an OAE, the deposition of sediments is associated with rapid changes in redox conditions from dysoxic to sulphidic conditions.
In summary, deep-water settings during the LASE in the Montagne Noire were relatively more oxygenated than those in other areas, such as the Kowala section in the Holy Cross Mountains in Poland and the Kronhofgraben section in the Carnic Alps in Austria. During the LASE, deposition was connected with the global marine crenulata transgression caused by the deglaciation of Gondwana. This period involved elevated productivity and halted carbonate production, as evidenced by drastic transitions from the deposition of carbonate to black siliceous, often organic-rich sedimentation (see Siegmund et al. Reference Siegmund, Trappe and Oschmann2002; Kaiser et al. Reference Kaiser, Becker, Steuber and Aboussalam2011; Bábek et al. Reference Bábek, Kumpan, Kalvoda and Grygar2016; Rakociński et al. Reference Rakociński, Marynowski, Zatoń and Filipiak2021 a, Reference Rakociński, Książak, Pisarzowska and Marynowski2022). Similar to Bond et al. (Reference Bond, Wignall and Racki2004), who studied the anoxia record during the Kellwasser Events, we conclude that anaerobic conditions do not have a universal record in different areas.
5.c. Mercury as an indicator of volcanic activity
The LASE was associated with climate change (warming), which caused the deglaciation of Gondwana, and subsequently a global marine transgression. The transgression led to increases in productivity and the development of anoxic conditions, especially in the deep-shelf settings (Becker, Reference Becker and House1993 a; Siegmund et al. Reference Siegmund, Trappe and Oschmann2002; Becker et al. Reference Becker, Kaiser and Aboussalam2006; Bábek et al. Reference Bábek, Kumpan, Kalvoda and Grygar2016; Rakociński et al. Reference Rakociński, Marynowski, Zatoń and Filipiak2021 a, Reference Rakociński, Książak, Pisarzowska and Marynowski2022). Climate warming was confirmed by a decrease to 18 ‰ of δ18Oapatite during the LASE (for detail see figs 11, 13 in Buggisch et al. Reference Buggisch, Joachimski, Sevastopulo and Morrow2008; Chen et al. Reference Chen, Chen, Qie, Huang, He, Joachimski, Regelous, Pogge von Strandmann, Liu, Wang, Montañez and Algeo2021), drastic facies changes and a maximum flooding marked by deposition of the Lower Alum Shale at the base of the mid-Tournaisian (Becker, Reference Becker and House1993 a; Siegmund et al. Reference Siegmund, Trappe and Oschmann2002; Kaiser et al. Reference Kaiser, Aretz, Becker, Becker, Königshof and Brett2015). However, the key question is on the primary factor that was responsible for environmental changes during the LASE. According to Siegmund et al. (Reference Siegmund, Trappe and Oschmann2002), eutrophication was connected to enhanced upwelling and an increased input of volcaniclastic nutrients. Volcanic activities increased during the mid-Tournaisian, and different styles of volcanic activity and types of volcanic centres are known (for details see Table 5 and discussion in Rakociński et al. Reference Rakociński, Książak, Pisarzowska and Marynowski2022). These activities and centres encompass e.g. the carbonatite Kola-Dnieper Igneous Province (Motuza et al. Reference Motuza, Šliaupa and Timmerman2015), subduction-related arc volcanism (e.g. Acquafredda et al. Reference Acquafredda, Lorenzoni and Lorenzoni1991; Lardeaux et al. Reference Lardeaux, Schulmann, Faure, Janoušek, Lexa, Skrzypek, Edel, Stípska, Schulmann, Martínez Catalán, Lardeaux, Janoušek and Oggiano2014), extensional and rift-related volcanism (Alexandre et al. Reference Alexandre, Chalot-Prat, Saintot, Wijbrans, Stephenson, Wilson, Kitchka and Stovba2004; Bryan et al. Reference Bryan, Allen, Holcombe and Fielding2004; Göncüoglu et al. (Reference Göncüoğlu, Türkmenoğlu, Bozkaya, Ünlüce-Yücel, Okuyucu and Yilmaz2016) and basaltic intraplate volcanism (e.g. Nesbor, Reference Nesbor2004).
Table 5. Examples of volcanic and/or magmatic activities around the Devonian–Mississippian and the mid-Tournaisian interval

Therefore, many volcanic and magmatic centres should be responsible for environmental turnovers at the local scale such as e.g. volcanic fertilization causing an increase in productivity, reduction of carbonate production, as well as a long-term massive CO2 release, leading to the greenhouse effect and global warming as postulated by Rakociński et al. (Reference Rakociński, Marynowski, Zatoń and Filipiak2021 a). All these factors would ultimately lead to the development of anoxic conditions and a resultant biotic crisis.
Recently, Hg anomalies have been widely used as tracers of massive volcanism in the sedimentary record (Sanei et al. Reference Sanei, Grasby and Beauchamp2012; Percival et al. Reference Percival, Ruhl, Hesselbo, Jenkyns, Mather and Whiteside2017; Racki et al. Reference Racki, Rakociński, Marynowski and Wignall2018 b; Jones et al. Reference Jones, Percival, Stokke, Frieling, Mather, Riber, Schubert, Schultz, Tegner, Planke and Svensen2019; Rakociński et al. Reference Rakociński, Marynowski, Zatoń and Filipiak2021 a, Reference Rakociński, Książak, Pisarzowska and Marynowski2022; Shen et al. Reference Shen, Chen, Algeo, Feng, Yu, Xu, Xu, Lei, Planavsky and Xie2021). This is because volcanic eruptions and submarine volcanic and hydrothermal activities are the main natural sources of mercury in recent and ancient environments, and are reflected by Hg spikes in sedimentary rocks (e.g. Pyle & Mather, Reference Pyle and Mather2003; Grasby et al. Reference Grasby, Them, Chen, Yin and Ardakani2019; Lim et al. Reference Lim, Kim, Kim, Jeong and Kim2020; Edwards et al. Reference Edwards, Kushner, Outridge and Wang2021).
Rakociński et al. (Reference Rakociński, Marynowski, Zatoń and Filipiak2021 a) for the first time described Hg anomalies during the LASE in the Polish part of Laurussia in the Holy Cross Mountains. The enrichments detected in the Zaręby Beds, the LASE age equivalent, were moderate with maximum values reaching 240 ppb (the background values in older Radlin Beds were c. 20 ppb). Additionally, numerous ash layers and zircon-enriched cryptotephra with high Zr contents reaching up to 4433.5 ppm (avg. Zr(EF) = 5.86, with maximum values of Zr(EF) = 32.65) were documented in the Kowala section. These results indicate significant explosive eruption during this episode in that part of Laurussia (Rakociński et al. Reference Rakociński, Marynowski, Zatoń and Filipiak2021 a). The potential source of the ash layers and cryptotephra could be within-plate explosive rift-related volcanism, such as ash layers in the Tournaisian found at the Zonguldak terrane in Turkey (cf. Göncüoglu et al. Reference Göncüoğlu, Türkmenoğlu, Bozkaya, Ünlüce-Yücel, Okuyucu and Yilmaz2016). A very large anomalous Hg spike has recently been found in the Carnic Alps with maximum values reaching 3650 ppb, generally >1000 ppb (Rakociński et al. Reference Rakociński, Książak, Pisarzowska and Marynowski2022). However, no traces of Zr enrichments, ash layers or cryptotephra have been detected. Therefore, according to Rakociński et al. (Reference Rakociński, Książak, Pisarzowska and Marynowski2022), very large Hg anomalies in the Carnic Alps were connected to regional submarine (arc) volcanism and hydrothermal activity, which was associated with the collision of the peri-Gondwanan terranes with Laurussia during the early phase of the Variscan orogeny (see Stampfli et al. Reference Stampfli, von Raumer, Borel, Martinez Catalán, Hatcher, Arenas and Diaz Garcia2002, Reference Stampfli, von Raumer, Wilhem, Gutierrez-Marco, Rabano and García-Bellido2011; Arboit et al. Reference Arboit, Chew, Visoná, Massironi, Sciascia, Benedetti and Rodani2008; von Raumer & Stampfli, Reference von Raumer and Stampfli2008).
Our data from Montagne Noire could confirm the suggestions presented above, because the Hg content increase, in comparison to the limestones (Figs 10, 11), generally reached >100 ppb with three Hg spikes >500 ppb in the PDLS (Fig. 10) in the LASE interval. The low concentrations of Zr in the limestones (with avg. Zr(EF) = 1.01 in the PDLS and avg. Zr(EF) = 1.20 in the CDT, respectively; see Tables 3, 4) and depletion of Zr in the radiolarian cherts (with avg. Zr(EF) = 0.86 in both sections; see Tables 3, 4), reflect the lack of higher pyroclastic input typical of explosive silicic volcanism. The very low TOC values in the lower part of the PDLS section (<0.2 wt %), where high Hg spikes occur, imply that organic matter could not control the Hg concentration spikes. It is also confirmed by very low Hg versus TOC correlation in the investigated sections, and by relatively low correlation of Hg versus TS. Notably, Hg versus Al2O3 reflected no correlations, indicating that Hg enrichments were not dependent on clay fraction and terrigenous input. These data partly correspond to the results for modern and ancient sediments (Shen et al. Reference Shen, Feng, Algeo, Liu, Zhou, Wei, Liu, Them, Gill and Chen2020), especially in terms of the poor or moderate correlation of Hg with Al and TS reflecting limited influence of sulphides and clay fractions on Hg concentrations. Data for the PDLS section exhibit a moderate correlation between Hg and TS; however, the impoverishment of TS in both sections also excludes the sulphide fraction as a potential host of mercury. It is indicative of a volcanic source for Hg in the investigated sections. The lower values of Hg enrichments in the Montagne Noire region imply that this area was situated further away from the source of mercury expulsion than the Carnic Alps, but closer than the Holy Cross Mountains (Fig. 14).
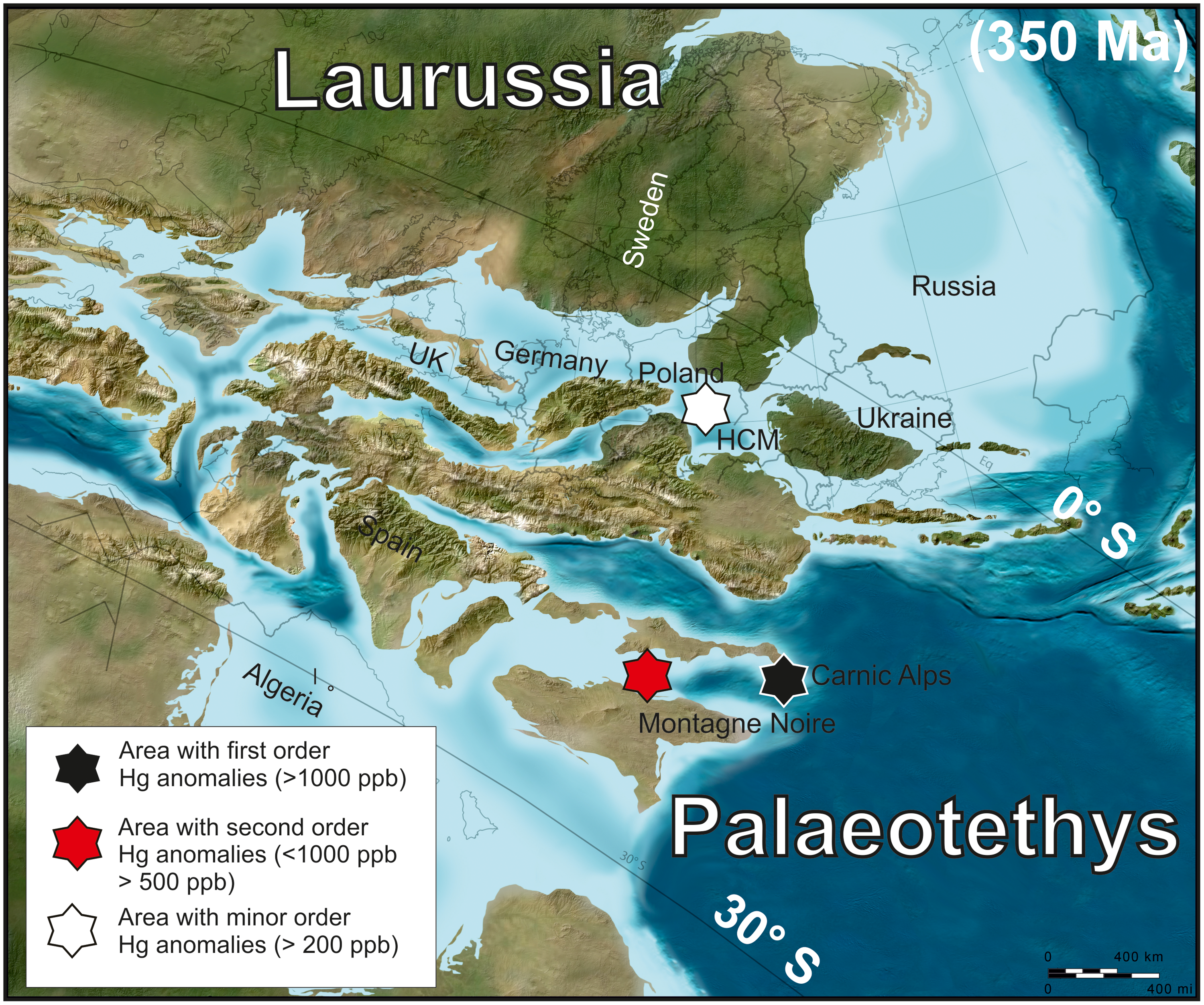
Fig. 14. Location of the LASE sections studied for Hg abundances, ranked in terms of their enrichments. Palaeogeography of Europe during the Tournaisian Age ∼350 Ma (Blakey, Reference Blakey2012) showing the location of the Montagne Noire area (orange star), Holy Cross Mountains (yellow star) and Carnic Alps (red star); © 2012 Colorado Plateau Geosystems Inc. Compare with reconstructions of the Variscan domain for the early Famennian and late Tournaisian after Stampfli et al. (Reference Stampfli, Hochard, Vérard, Wilhem and von Raumer2013, fig. 8).
Although a lot of mid-Tournaisian active volcanic centres are known, precise dating of some of them is at least problematic (see Table 5). Many of them encompass interesting mid-Tournaisian time intervals; however, some of them embrace longer time intervals and the time of their main pulses is also unclear. Therefore, deciphering the source area is not easy. For example, a potential source of Hg may be the NE part of the French Massif Central and Vosges Mountains (Moldanubian Zone) where Upper Devonian/Mississippian volcano-sedimentary rocks were deposited as a result of the development of a short-lived magmatic arc in relation to oceanic subduction (Lardeaux et al. Reference Lardeaux, Schulmann, Faure, Janoušek, Lexa, Skrzypek, Edel, Stípska, Schulmann, Martínez Catalán, Lardeaux, Janoušek and Oggiano2014). Another probable cause of the observed Hg spikes in the Montagne Noire could be regional submarine arc volcanism and hydrothermal activity connected with the collision of the peri-Gondwanan Palaeo-Adria terranes and probably Armorican Terrane Assemblage and/or Noric terrane (for details see discussion in Rakociński et al. Reference Rakociński, Książak, Pisarzowska and Marynowski2022 and references therein). Regardless of the potential source of Hg, the overlap of many different styles of volcanic activities could have been responsible for significant short-term changes on a regional scale and climate warming on a long-term scale, which drove the deglaciation of Gondwana and the resultant marine transgression inducing biotic turnover known as the LASE. The middle Palaeozoic was a time of extensive geotectonic Variscan turnover, which began press-pulse volcanic-tectonic activity (see e.g. Murphy et al. Reference Murphy, Gutierrez-Alonso, Nance, Fernandez-Suarez, Keppeie, Quesada, Strachan and Dostal2006; von Raumer & Stampfli, Reference von Raumer and Stampfli2008). These activities could have been a source of many metals which on the one hand stimulated primary productivity, and on the other hand, had toxic effects on the biosphere.
Therefore, volcanism could have been the driver of increased productivity, global warming and, consequently, oxygen-depletion during many marine and oceanic anoxic events and biotic crises (e.g. Jenkyns, Reference Jenkyns2010; Rakociński et al. Reference Rakociński, Książak, Pisarzowska and Marynowski2022). The results of the present study imply that not only activity of Large Igneous Provinces but generally various styles of volcanic activity, often overlapping, related to different geotectonic realms could have caused climatic changes and anoxic events (see Rakociński et al. Reference Rakociński, Marynowski, Zatoń and Filipiak2021 a, Reference Rakociński, Książak, Pisarzowska and Marynowski2022). Therefore, the underappreciated impact of arc volcanism and hydrothermal activities (oceanic intraplate volcanism) has recently been exemplified during climatic changes (e.g. McKenzie et al. Reference McKenzie, Horton, Loomis, Stockli, Planavsky and Lee2016; Lu et al. Reference Lu, Wang, Yang, Shao and Hilton2021) and other biotic crises, such as the Ordovician–Silurian (Yang et al. Reference Yang, Hu, Wang, Jiang, Yao, Sun, Huang and Zhu2019), end-Frasnian (Racki, Reference Racki2020), end-Devonian (Rakociński et al. Reference Rakociński, Pisarzowska, Corradini, Narkiewicz, Dubicka and Abdiyev2021 b) and end-Permian (Shen et al. Reference Shen, Chen, Algeo, Feng, Yu, Xu, Xu, Lei, Planavsky and Xie2021).
Many researchers have recently postulated that the main factor responsible for OAE development was an abrupt rise in temperature induced by the rapid influx of CO2 into the atmosphere connected with increased volcanic activity and/or methane thermogenic release (e.g. Jenkyns, Reference Jenkyns2010). However, the causes of particular anoxic events during Phanerozoic time were variable. They remain uncertain and are still being debated, because their consequences were different (e.g. Kuhnt et al. Reference Kuhnt, Holburn and Moullade2011; Cheng et al. Reference Cheng, Elrick and Romaniello2020; Chen et al. Reference Chen, Chen, Qie, Huang, He, Joachimski, Regelous, Pogge von Strandmann, Liu, Wang, Montañez and Algeo2021). For example, during a little younger, mid-Tournaisian carbon isotopic excursion (TICE), the development of anoxia was caused by different triggers than the LASE. The TICE was one of the largest perturbations in the global carbon cycle and organic carbon burial episodes in the Phanerozoic, which drove the late Palaeozoic cooling and glaciation (Saltzman et al. Reference Saltzman, González and Lohmann2000; Cheng et al. Reference Cheng, Elrick and Romaniello2020; Chen et al. Reference Chen, Chen, Qie, Huang, He, Joachimski, Regelous, Pogge von Strandmann, Liu, Wang, Montañez and Algeo2021). The postulated causes of this anoxic event include increased productivity connected with the supply of riverine nutrients and upwelling, as well as the expansion of terrestrial flora, which enhanced chemical and physical weathering (Saltzman et al. Reference Saltzman, González and Lohmann2000; Yao et al. Reference Yao, Qie, Luo, Liu, Algeo, Bai, Yang and Wang2015; Liu et al. Reference Liu, Algeo, Qie and Saltzman2019; Cheng et al. Reference Cheng, Elrick and Romaniello2020; Chen et al. Reference Chen, Chen, Qie, Huang, He, Joachimski, Regelous, Pogge von Strandmann, Liu, Wang, Montañez and Algeo2021). In contrast to other middle Palaeozoic events such as the Late Devonian Kellwasser and Hangenberg crises, and mid-Tournaisian LASE event (Saltzman et al. Reference Saltzman, González and Lohmann2000; Kaiser et al. Reference Kaiser, Aretz, Becker, Becker, Königshof and Brett2015; Racki, Reference Racki2020), the TICE does not show a close coincidence with extinctions.
6. Conclusions
Based on changes in such redox-sensitive trace elements such as U, Mo and V and Corg/P, U/Th and V/Cr ratios, as well as pyrite framboid analyses, a high-resolution record of changes in redox conditions was for the first time documented for the Tournaisian sediments of the Montagne Noire.
The lower part of the black radiolarian cherts in the study area was deposited under periodic dysoxic to anoxic conditions in the bottom and water column, and constitutes an equivalent of the mid-Tournaisian anoxic event referred to as the LASE. Deep-water settings recorded in the Montagne Noire were relatively better oxygenated than in other sites, such as the Kowala section in Poland and Kronhofgraben section in the Carnic Alps in Austria. The results obtained in the present study show that on a regional scale, the LASE in a pelagic setting was not uniform.
Large Hg spikes >500 ppb were connected with regional submarine volcanism and hydrothermal activities most probably connected with the collision of the peri-Gondwanan Palaeo-Adria terranes and probably the Armorican Terrane Assemblage and/or Noric terrane or a short-lived magmatic arc lying in the NE part of the French Massif Central and Vosges Mountains (Moldanubian Zone).
Numerous regional magmatic centres were potentially responsible for local drastic changes and the productivity increase on a global scale. These likely caused the climatic turnover, Gondwana deglaciation, global transgression and development of anoxia in many parts of the world during the mid-Tournaisian anoxic event.
Supplementary material
To view supplementary material for this article, please visit https://doi.org/10.1017/S0016756822001297
Acknowledgements
We thank Katarzyna Kołtonik (Institute of Nuclear Physics PAS in Krakow) for help during our field works in 2016. We thank Renata Fikier and Iwona Milińska for Hg measurements (both University of Silesia in Katowice). Two anonymous reviewers and Emese Bordy, the journal editor, are thanked for useful remarks, criticism and constructive comments which helped to improve the final version of the manuscript. We would also like to thank Editage (www.editage.com) for language correction of the text. This study was supported by the National Science Centre in Poland (Grant UMO-2014/15/B/ST10/03705 to MR).