Introduction
Mazorite, ideally Ba3(PO4)2, was discovered as an accessory phase in coarse-grained rankinite paralava embedded in massive gehlenite-rich pyrometamorphic rocks of the Hatrurim Complex in Israel (31°11'09“N, 35°16'27”E). The same mineral (described as a P-analogue of gurimite) was also recently detected in the carbonate–silicate xenolith of the Bellerberg volcano in Germany (Juroszek and Ternes, Reference Juroszek and Ternes2022).
Mazorite as the P-analogue of gurimite Ba3(VO4)2 (Galuskina et al., Reference Galuskina, Galuskin, Vapnik, Prusik, Stasiak, Dzierżanowski and Murashko2017), and Ba-analogue of tuite Ca3(PO4)2 (Xie et al., Reference Xie, Minitti, Chen, Mao, Wang, Shu and Fei2004), is isostructural to palmierite K2Pb(SO4)2 (Tissot et al., Reference Tissot, Rodriguez, Sipola and Voigt2001) and kalistrontite K2Sr(SO4)2 (Kemp et al., Reference Kemp, Rushton, Horstwood and Nénert2018). Its relation to the minerals of the recently accepted palmierite supergroup is presented in the second paper (Juroszek et al., Reference Juroszek, Krüger, Krüger and Galuskina2023).
In addition to minerals, numerous isostructural synthetic compounds are known. The inorganic crystal structure database lists 99 entries with the palmierite-type structure (data for May 2023)(of note: Zachariasen, Reference Zachariasen1948; Süsse and Buerger, Reference Süsse and Buerger1970; Sugiyama and Tokonami, Reference Sugiyama and Tokonami1990; Thompson et al., Reference Thompson, Xie, Zhai, Downs and Yang2013; Tsyrenova et al., Reference Tsyrenova, Pavlova, Solodovnikov, Popova, Kardash, Stefanovich, Gudkova, Solodovnikova and Lazoryak2016; Bismayer et al., Reference Bismayer, Mihailova and Angel2017). In general, the synthetic Ba and Sr orthophosphates are isomorphous with orthovanadates and orthoarsenates of the same alkaline earth elements (Zachariasen, Reference Zachariasen1948; Süsse and Buerger, Reference Süsse and Buerger1970).
In materials science, Ba3(PO4)2 is an important phosphor material characterised by good luminescence efficiency and colour purity. The crystal structure is quite flexible and can accommodate different luminescent ions, such as rare-earth elements (Tāle et al., Reference Tāle, Kūlis and Kronghauz1979; Mu and He, 2012; Tong et al., Reference Tong, Liang, Yan, Wang and Li2015). Previous publications show that barium orthophosphate is a host material characterised by the ability to form visible-emitting phosphors doped with metal and rare-earth ions e.g. Ni2+, Mn2+,3+,5+, Ce3+, Eu2+,3+, Yb2+, Sm3+ and Tb3+ (Lagos, Reference Lagos1970; Tāle et al., Reference Tāle, Kūlis and Kronghauz1979; Cao et al., Reference Cao, Yu, Sun, Cao and Qiu2014; Tong et al., Reference Tong, Liang, Yan, Wang and Li2015). Furthermore, a novel magnetic absorbent (BPF), a composite of Ba3(PO4)2 nanoflakes and Fe3O4 microspheres, could be an interesting material for removing organic dyes, like methyl blue, from polluted water, due to their good absorption and easy magnetic separation abilities (Zhang et al., Reference Zhang, Yin, Lan and Zhang2016).
Mazorite was approved by the Commission on New Minerals, Nomenclature and Classification of the International Mineralogical Association (IMA2022–022, Juroszek et al., Reference Juroszek, Galuskina, Krüger, Krüger, Vapnik and Galuskin2022), symbol Mzo. The type material was deposited in the mineralogical collection of the Natural History Museum, Bernastrasse 15, CH-3005, Bern, Switzerland (inventory number NMBE 44297).
The name mazorite is chosen in honour of Professor Emanuel Mazor (1933–2021), who worked at the Department of Environmental Sciences and Energy Research, Weizmann Institute of Science in Israel. Professor Mazor was a geologist, head of a radioactive study at the Israel Atomic Energy Commission (1958–1972), and author and co-author of many publications. He was also the author of one of the earliest studies of the Hatrurim Complex in Israel (see Gross et al., Reference Gross, Mazor, Sass and Zak1967). Emanuel Mazor was involved in persuading the government to turn the Makhtesh Ramon area in the Negev Desert into a national park. Thanks to his efforts, this park now includes public education and scientific research centres.
The present paper provides a detailed description of the new mineral mazorite from the type locality of the Hatrurim Complex in Israel. In addition, on the basis of single-crystal X-ray diffraction work, the first structural data for P-rich gurimite are reported. Furthermore, the results obtained are compared to chemical and spectroscopic analyses performed on mazorite found in xenoliths of the Bellerberg volcano, Germany.
Methods of investigation
The chemical composition, crystal morphology, and optical properties of holotype mazorite and associated minerals were examined using an optical microscope and a Phenom XL analytical scanning electron microscope (Institute of Earth Sciences, Faculty of Natural Sciences, University of Silesia, Sosnowiec, Poland).
Electron microprobe analyses (EMPA) for the holotype specimen from the Hatrurim Complex were carried out on CAMECA SX100 (Polish Geological Institute, National Research Institute, Warsaw, Poland) at 15 kV and 20 nA using the following lines (and standards): NaKα (NaCl); KKα, AlKα (orthoclase); BaLβ (baryte); SrLα (celestine); CaKα, PKα (apatite); SiKα (zircon); VKα (V metal); SKα (chalcopyrite). The beam diameter was ~2 μm. In turn, quantitative chemical analyses of mazorite from the Bellerberg volcano, as well as Mo-bearing gurimite and minerals of the mazorite–gurimite series from the Hatrurim Complex, were performed using a CAMECA SX100 electron-microprobe (Faculty of Geology, University of Warsaw, Poland) at 15 kV and 10 nA with beam size ~2 μm. The following lines and standards were used: NaKα (albite); KKα, AlKα (orthoclase); BaLβ, SKα (baryte); SrLα (celestine); PbMα (crocoite); CaKα, SiKα (diopside); PKα (YPO4/LaPO4); FeKα (Fe2O3); VKα (V2O5); CrKβ (Cr2O3); MoLα (molybdenite).
The Raman spectra of mazorite from two localities and spectra of phases belonging to the mazorite–gurimite mineral series were recorded on a WITec alpha 300R Confocal Raman Microscope (Institute of Earth Sciences, Faculty of Natural Sciences, University of Silesia, Sosnowiec, Poland) equipped with an air-cooled solid laser 488 nm and a CCD camera operating at –61°C. Laser radiation was coupled to a microscope through a single-mode optical fibre with a diameter of 3.5 μm. An air Zeiss objective (L.D. EC Epiplan-Neofluan DIC-100/0.75NA) was used. The scattered Raman light was focused by an effective Pinhole size of ~30 μm and a monochromator with a grating of 1800 mm–1. The power of the laser at the sample position was 40 mW, with 5 s integration time and an accumulation of 20 scans over 3 cm–1 resolution. The monochromator was calibrated using the Raman scattering line of a silicon plate (520.7 cm–1). The spectra were processed using the GRAMS Spectracalc software package (Galactic Industries Corporation, NH, USA). The Raman bands were fitted using a Gauss–Lorentz cross-product function with the minimum number of component bands used for the fitting process.
Single-crystal X-ray diffraction (SC-XRD) experiments on mazorite and the P-rich gurimite were carried out using synchrotron radiation. The diffraction experiments were performed at the X06DA beamline of the Swiss Light Source (Paul Scherrer Institute, Villigen, Switzerland). The wavelength was tuned to 0.70848 Å, and the PRIGo goniometer was used to position and rotate the crystal. Diffracted intensities were recorded with the Pilatus-2M-F detector, placed at a distance of 90 mm from the crystal, and vertically shifted by 55 mm off-centre to increase the data resolution. A total of 3600 (mazorite) / 1800 (P-rich gurimite) frames were recorded using a fine-sliced (0.1/0.2) ω-scan at 0.1 s per frame. The CrysAlisPRO software package (Rigaku Oxford Diffraction) was used to process the data.
The unit-cell dimensions and the space group of the mazorite and P-rich gurimite correspond to synthetic Ba3(PO4)2. The structures were refined from atomic coordinates reported by Sugiyama and Tokonami (Reference Sugiyama and Tokonami1990). The accurate electron densities at the cation sites were determined using the neutral atomic scattering factors in combination with a refinement of the individual site occupancies. The refinement of the site population of cations confirmed the partial substitution of Ba by K and P by V, matching the microprobe analysis data. More details concerning data collection conditions and crystal structure refinement parameters are reported in Table 1, atom coordinates, occupancies, and equivalent displacement parameters are presented in Table 2, and the crystallographic information files (cif) have been deposited with the Principal Editor of Mineralogical Magazine and are available as Supplementary material (see below).
Table 1. Parameters for X-ray data collection and crystal-structure refinement for mazorite and P-rich gurimite.

* w =1/(σ2(F)+(0.0001F 2)
Table 2. Atom coordinates (x,y,z), occupancies and equivalent displacement parameters (U eq, Å2) of mazorite and P-rich gurimite.

Occurrence, general appearance and mineral association
Geological settings
The unique Hatrurim Complex, well-known for the discovery of many new minerals, is composed of high-temperature low-pressure pyrometamorphic rocks formed as a result of combustion metamorphism, which are distributed on both sides of the Dead Sea Transform Fault in Israel, Palestine (West Bank) and Jordan (Gross, Reference Gross1977; Burg et al., Reference Burg, Starinsky, Bartov and Kolodny1991; Geller et al., Reference Geller, Burg, Halicz and Kolodny2012; Novikov et al., Reference Novikov, Vapnik and Safonova2013). Pyrometamorphic clinker-like rocks are represented mainly by larnite, gehlenite and spurrite-bearing rocks and are usually altered, veined and cracked by the products of their low-temperature transformations (Gross, Reference Gross1977; Burg et al., Reference Burg, Starinsky, Bartov and Kolodny1991; Novikov et al., Reference Novikov, Vapnik and Safonova2013; Galuskina et al., Reference Galuskina, Vapnik, Lazic, Armbruster, Murashko and Galuskin2014; Kolodny et al., Reference Kolodny, Burg, Geller, Halicz and Zakon2014). The presence of different types of paralava within the pyrometamorphic rock of the Hatrurim Complex indicated a local intensification of combustion processes that led to the partial or complete melting of protolith rocks (Sokol et al., Reference Sokol, Novikov, Vapnik and Sharygin2007, Reference Sokol, Novikov, Zateeva, Vapnik, Shagam and Kozmenko2010; Vapnik et al., Reference Vapnik, Sharygin, Sokol and Shagam2007; Grapes, Reference Grapes2011). Paralavas of the Hatrurim Complex, whose formation is determined by combustion temperature and the protolith composition, occur as lenses or dyke-like bodies of various sizes within the host rocks and exhibit fluidal vesicular textures (Vapnik et al., Reference Vapnik, Sharygin, Sokol and Shagam2007; Grapes, Reference Grapes2011). Some high-temperature minerals recognised in paralava suggest that the temperature could have reached more than 1000°C (Sokol et al., Reference Sokol, Novikov, Vapnik and Sharygin2007; Vapnik et al., Reference Vapnik, Sharygin, Sokol and Shagam2007). Despite many years of research on this geological unit, the origin of the Hatrurim Complex is still uncertain. More detailed information about the main hypotheses can be found in previous publications (Sokol et al., Reference Sokol, Novikov, Zateeva, Vapnik, Shagam and Kozmenko2010; Geller et al., Reference Geller, Burg, Halicz and Kolodny2012; Novikov et al., Reference Novikov, Vapnik and Safonova2013; Galuskina et al., Reference Galuskina, Vapnik, Lazic, Armbruster, Murashko and Galuskin2014).
The mazorite holotype location (31°11'09“N, 35°16'27”E) is a well-known geological area located in the upper reaches of a tributary of the Wadi Halamish in the Hatrurim Basin. This dry valley runs for several kilometres along the road from the Arad to the Dead Sea, and the pyrometamorphic rocks that occur there are the sources of numerous new minerals. Over a few years, several Fe, Ni and Ca phosphides and phosphates (Britvin et al., Reference Britvin, Murashko, Vapnik, Polekhovsky, Krivovichev, Vereshchagin, Vlasenko, Shilovskikh and Zaitsev2019, Reference Britvin, Murashko, Vapnik, Polekhovsky, Krivovichev, Vereshchagin, Shilovskikh and Krzhizhanovskaya2020, Reference Britvin, Murashko, Krzhizhanovskaya, Vapnik, Vlasenko, Vereshchagin, Bocharov and Vasilev2021), as well as new sulfide (Galuskina et al., Reference Galuskina, Krüger, Galuskin, Krüger, Vapnik, Banasik, Murashko, Agakhanov and Pauluhn2019) or zeolite phases (Skrzyńska et al., Reference Skrzyńska, Cametti, Galuskina, Vapnik and Galuskin2023) have been identified and described at this locality. Morphologically, the type locality is a hill covered by dark-burned pyrometamorphic rocks (Fig. 1a), represented mainly by fine-grained massive gehlenite-rich rocks (hornfelses) embedded in hydrothermally altered low-temperature rocks. Some of the collected samples contain coarse-grained fragments of paralava, in which mazorite was detected (Fig. 1b).

Figure 1. (a) The holotype locality: a hill covered by pyrometamorphic rocks of the Hatrurim Complex and (b) pyrometamorphic rocks represented by fine-grained massive gehlenite-rich rocks with dark fragments of coarse-grained paralava.
Mineral association, physical and optical properties of mazorite
Mazorite from the Hatrurim Complex (Hatrurim Basin outcrop) was found in small mineral aggregates (enclaves) of a coarse-grained paralava (Fig. 2). The presence of enclaves enriched with incompatible elements such as Ba, V, P, U, S, Ti and Nb is a distinctive feature that characterises the paralava (Krzątała et al., Reference Krzątała, Krüger, Galuskina, Vapnik and Galuskin2020). The host rock comprises mainly rankinite, fluorapatite, minerals of the gehlenite–alumoåkermanite–åkermanite, schorlomite–andradite series and kalsilite. Occasionally, wollastonite, larnite–flamite, magnesioferrite, magnetite, perovskite and Si-rich perovskite are observed. Within the analysed samples, small enclaves were filled with Ba-minerals, represented by mazorite, zadovite, Si-rich zadovite, celsian, hexacelsian, bennesherite, sanbornite, walstromite, fresnoite, gurimite, alforsite, fluoralforsite, barioferrite, Mn-bearing ferrite, baryte and a potentially new mineral Ca2BaMgSi2O8 as well as Ba–Ti–Nb and Ca–U–Si phases (Figs 2, 3a). Highly hydrated Ca-silicates, minerals of the tobermorite group, tacharanite and afwillite are secondary minerals that have been recognised in the paralava.
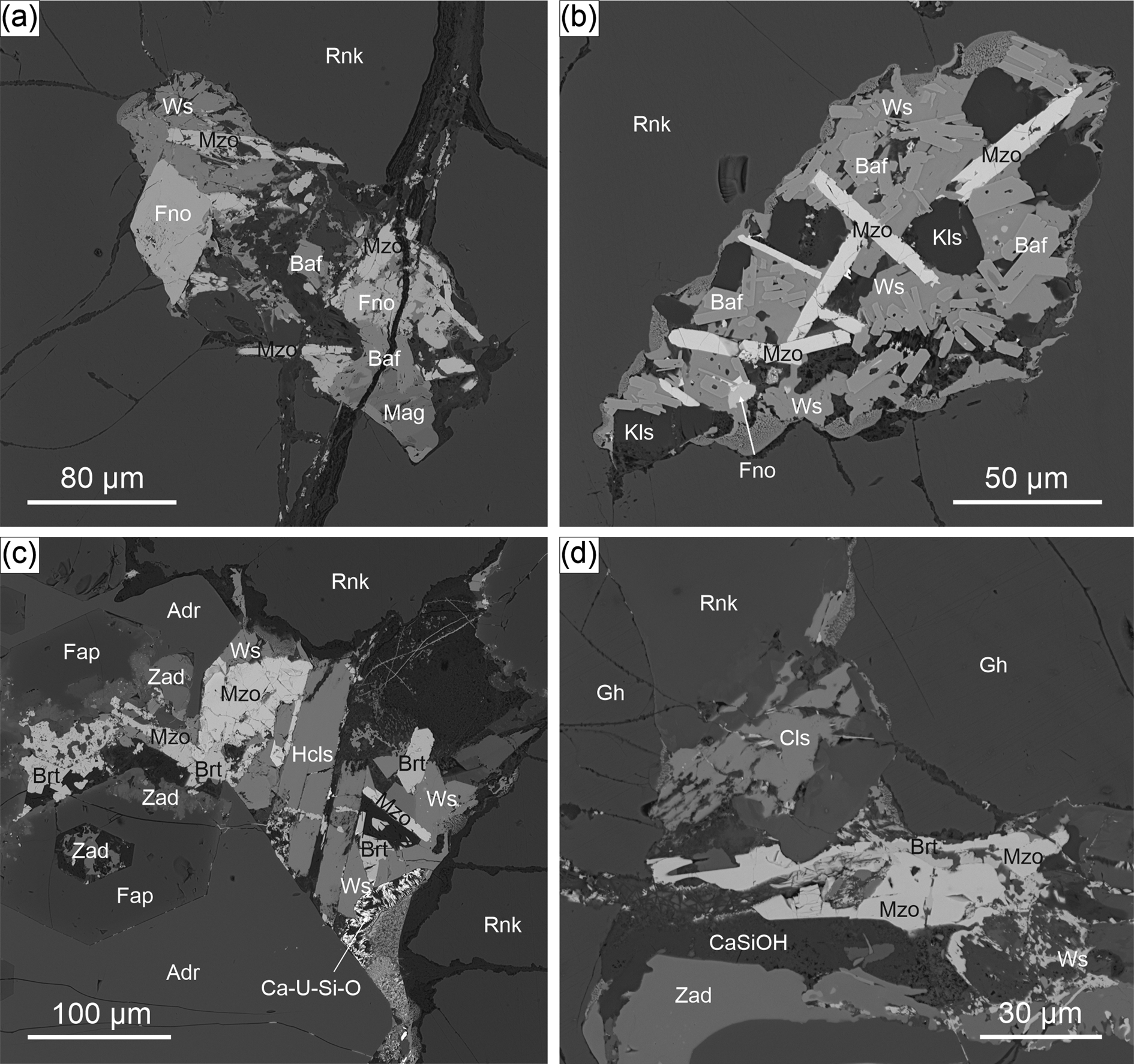
Figure 2. Small enclaves filled with rare Ba mineralisation within the gehlenite–rankinite paralava: (a,b) elongated flattened crystals of mazorite in association with other Ba-bearing minerals; and (c,d) aggregates of mazorite with baryte overgrowths. BSE (back-scattered electron) images. Symbols mostly from Warr (Reference Warr2021): Adr – andradite; Baf – barioferrite; Brt – baryte; CaSiOH – Ca-hydro silicates; Ca-U-Si-O – CaUSiO phase; Cls – celsian; Fap – fluorapatite; Fno – fresnoite; Gh – gehlenite; Hcls – hexacelsian; Kls – kalsilite; Mag – magnetite; Mzo – mazorite; Rnk – rankinite; Ws – walstromite; Zad – zadovite.

Figure 3. (a) BSE image of mazorite and associated minerals within the gehlenite–rankinite paralava. (b) Elongate plate-like crystals of mazorite in transmitted light and (c) under crossed polarisers. Symbols as in Fig. 2.
Mazorite forms very thin ~15 μm and elongated individual plate-like crystals up to 70–100 μm in length. (Figs 2a,b; 3a). Rarely, some ~100 μm large aggregates of mazorite grains (up to 20 μm) were observed (Fig. 2c). In these cases, mazorite–baryte intergrowths are noted (Fig. 2c,d). The mineral appears as colourless crystals with a vitreous lustre and white streak. It shows good cleavage on (001), nonetheless, parting or twinning was not observed. The tenacity is brittle, and the fracture is uneven or irregular.
In transmitted light, mazorite is transparent and non-pleochroic (Fig. 3b). Under crossed polarisers, strong anisotropy and parallel extinction were observed (Fig. 3c). Optically, mazorite is uniaxial (+), with ω = 1.760(3), ɛ = 1.766(3) (λ = 589 nm).
Micro-indentation hardness measurements VHN100 = 461 kg/ mm2, mean of seven analyses (range from 452 to 467 kg/mm2) corresponds to a hardness of ~4.5 on the Mohs scale. The tiny crystal size did not allow for the selection of adequate grains for an accurate density measurement, therefore, the density was calculated as 4.81 g⋅cm–3 based on the empirical formula and unit-cell volume refined from the XRD data. For the ideal formula, the Gladstone–Dale compatibility index (Mandarino, Reference Mandarino1989) is 1 – (K p/K c) = 0.000 (superior).
Mazorite was also found in a carbonate–silicate xenolith from the Caspar quarry, Bellerberg volcano, Eifel, Germany. This locality is well-known for various thermally metamorphosed xenoliths embedded in basaltic lava, characterised by mineralogical diversity, and famous for new mineral findings (Irran et al., Reference Irran, Tillmanns and Hentschel1997; Mihajlovic et al., Reference Mihajlovic, Lengauer, Ntaflos, Kolitsch and Tillmanns2004; Chukanov et al., Reference Chukanov, Britvin, Van, Möckel and Zadov2012; Galuskin et al., Reference Galuskin, Krüger, Krüger, Blass, Widmer and Galuskina2016; Juroszek et al., Reference Juroszek, Krüger, Galuskina, Krüger, Jeżak, Ternes, Wojdyla, Krzykawski, Pautov and Galuskin2018b; Juroszek and Ternes, Reference Juroszek and Ternes2022). More geological information concerning this locality can be found in Hentschel (Reference Hentschel1987).
In the xenolith sample, mazorite was detected as an inclusion inside a bennesherite Ba2Fe2+Si2O7 crystal. The size of an individual and irregular grain of mazorite did not exceed 15 μm. Mazorite identified in the Bellerberg volcano is a member of unusual Ba-mineralisation, comprising accessory minerals such as bennesherite, walstromite, fresnoite, zadovite and celsian. Ba-minerals have crystallised in small cracks and veins between the rock-forming minerals, mostly wollastonite, nepheline, gehlenite and minor alumoåkermanite (Juroszek and Ternes, Reference Juroszek and Ternes2022).
Results
Chemical composition
The results of the electron microprobe analyses of holotype mazorite are presented in Table 3. These data were compared with the EMPA results of mazorite from the Bellerberg volcano and mineral phases belonging to the mazorite–gurimite series of some Hatrurim Complex outcrops. The empirical formula of the holotype mazorite calculated on the basis of 8O is (Ba2.69K0.22Na0.04Ca0.02Sr0.01)Σ2.98(P1.16V0.57S0.24Al0.04Si0.03)Σ2.04O8, which leads to the simplified formula (Ba,K)3[(P,V,S)O4]2. The end-member formula Ba3(PO4)2 corresponds to 76.42 wt.% of BaO and 23.58 wt.% of P2O5. Mazorite from the holotype locality (Hatrurim) might be considered a complex solid-solution consisting of the following end-members: 57% of mazorite, 28% of gurimite and 12% of K2Ba(SO4)2. The content of other components is ~3%. The composition of the mazorite sample from Bellerberg is closer to the end-member formula, with 86% of the end-member content. The empirical formula obtained from the two analyses is as follows: (Ba2.59Ca0.23Sr0.10K0.05Na0.05)Σ3.02(P1.76S0.10Si0.08Al0.04Fe0.04V0.02)Σ2.04O8. Mazorite from Bellerberg shows a lower content of Na+, K+ and S6+ compared to the Hatrurim samples. Furthermore, all Hatrurim samples show several times higher amounts of V5+ than the Bellerberg samples (Table 3).
Table 3. Chemical composition of minerals belonging to the mazorite–gurimite series.*

* S.D. = 1σ = standard deviation; n = number of analyses; n.d. = not detected.
The chemical composition of the minerals belonging to the mazorite–gurimite series from different outcrops in Hatrurim, Israel, is presented in Table 3. The JD50a and AM2 samples are closest to the intermediate phase with almost equal amounts of P2O5 and V2O5. In turn, the composition of gurimite from the ZZ2 and AM3 samples shows a significant increase of V5+ over P5+. Nevertheless, there is a considerable difference between them. ZZ2 indicated ~8 wt.% enrichment of MoO3, corresponding to 0.33 apfu. However, sample AM3, similar to AM2 and JD50, exhibits an increased SO3 content of ~3–4.5 wt.%. A higher amount of hexavalent constituents in the composition of the mazorite–gurimite mineral series is balanced by an increase in monovalent components, mainly K.
Raman spectroscopic data
The variations in the composition are reflected in the occurrence of different vibration modes related to the anionic groups in the Raman spectra of mazorite from the Hatrurim Complex and Bellerberg volcano (Fig. 4). However, both spectra exhibit similarities, especially in the bands assigned to the phosphate (PO4)3– groups. An intense Raman band at ~926 cm–1 related to the ν1 symmetric stretching vibrations of (PO4)3– was characteristic for both mazorite spectra (Fig. 4a,b). Asymmetric stretching ν3 modes are observed at 1037 and 1044 cm–1 in the spectrum of the Hatrurim (Fig. 4a) and the Bellerberg (Fig. 4b) specimens, respectively. The Raman bands at 412 cm–1 and 562 cm–1 correspond to the symmetric (ν2) and asymmetric (ν4) bending vibrations of the (PO4)3– groups in the spectrum of mazorite from the Hatrurim Complex (Fig. 4a). In turn, mazorite from the Bellerberg volcano exhibits the same vibrations as a band at 407 cm–1 with a shoulder at 420 cm–1 (ν2) and a band at 558 cm–1 (ν4) (Fig. 4b). The low-wavenumber region below 250 cm–1 is attributed to the external Ba–O vibrations.

Figure 4. Raman spectra of mazorite from (a) the holotype locality, Hatrurim, Israel and (b) Caspar quarry, Bellerberg volcano, Germany.
Additional bands observed in the mazorite spectra, as confirmed by chemical and structural investigations, came from (VO4)3–, (SO4)2– and (SiO4)4– groups, which can occupy the same tetrahedral site as the (PO4)3– groups in the mazorite structure. In both spectra, Raman bands at 982, 464/457 and 616 cm–1 correspond to ν1 symmetric stretching and ν2+ν4 bending vibrations of the sulfate groups, respectively. Mazorite from Hatrurim contains a significant amount of vanadium in the chemical composition. Therefore, Raman bands in the ranges ~330–370 cm–1 and 800–865 cm–1 are assigned to the bending (ν2+ν4) and stretching (ν1+ν3) vibrations of the (VO4)3– group (Fig. 4a). In the case of mazorite from Bellerberg, the vanadium content is negligible with respect to the P, S, and even Si content (Table 2). Thus, the Raman bands of relatively low intensities at 860 cm–1 and 345 cm–1 (Fig. 4b) can be ascribed to the symmetric stretching and bending modes of the (VO4)3– groups. The band at 825 cm–1 with a shoulder at 812 cm–1 is probably related to the ν1 symmetric stretching vibration of (SiO4)4– groups. Furthermore, the band at 954 cm–1 attributed to the ν3 asymmetric stretching vibration of the (SiO4)4– group may also overlap with the ν1 mode of (PO4)3–.
The results obtained are in good agreement with the published data. In Table 4, the Raman data for mazorite from Hatrurim and Bellerberg are correlated with the spectroscopic data of synthetic Ba3(PO4)2 (Zhai et al., Reference Zhai, Liu, Xue and Song2011) and gurimite from the type locality (Galuskina et al., Reference Galuskina, Galuskin, Vapnik, Prusik, Stasiak, Dzierżanowski and Murashko2017). The main difference between natural and synthetic barium orthophosphate is associated with the additional bands related to the (VO4)3–, (SiO4)4– and (SO4)2– vibrations, which are observed in the natural counterparts (Table 4). Furthermore, in the Raman spectrum of the synthetic Ba3(PO4)2, the presence of two bands assigned to the asymmetric stretching vibrations (ν3) at 1043 and 980 cm–1 is observed (Zhai et al., Reference Zhai, Liu, Xue and Song2011). For both natural mazorite specimens, only one band is related to these vibrations because the band at 982 cm–1 was ascribed to the ν1 of (SO4)2– according to the chemical composition results. Raman bands attributed to the vibrations of vanadate groups in mazorite confirm their frequencies in relation to the gurimite spectrum (Galuskina et al., Reference Galuskina, Galuskin, Vapnik, Prusik, Stasiak, Dzierżanowski and Murashko2017). However, their number and modification are distinguished as a result of the predominant phosphorus content compared to the gurimite spectrum. In gurimite, the band at 418 cm–1 was assigned to the ν4 of (VO4)3–, whereas it is more suitable for the ν2 of (PO4)3– (Table 3; Galuskina et al., Reference Galuskina, Galuskin, Vapnik, Prusik, Stasiak, Dzierżanowski and Murashko2017).
Table 4. Comparison of Raman band assignments for natural Ba3(PO4)2, its synthetic analogue and gurimite.

The existence of a mazorite–gurimite solid-solution is confirmed by chemical analyses (Table 3) and Raman spectroscopy. Their correlation allows us to interpret gradual band shifts in the spectra related to the substitution of P5+ and V5+ in this mineral series. The variations within the position of the stretching and bending modes of phosphate, vanadate and sulfate ions are shown in Fig. 5a–f. The observed shift for bands related to the ν1 (PO4)3– is ~6 cm–1: from 920 cm–1 for gurimite with 2% PO4 to the 926 cm–1 for the mazorite with 64% PO4 (Fig. 5a,f). The band related to the asymmetric stretching vibrations (ν3) is located at 1030 cm–1 in the spectrum of the almost intermediate phase (47% PO4; Fig. 5d) and shifts ~7 cm–1 to the higher wavenumber 1037 cm–1 for mazorite with 64% PO4 (Fig. 5f). For modes ascribed to the bending vibrations, some modifications, such as bands splitting and broadening were noted, primarily for ν2 of (PO4)3–. Splitting of these modes is observed for members with the PO4 constituent in the 35–60% range. The bands attributed to the asymmetric bending (ν4) remain unchanged and appear at ~562 cm–1 (Fig. 5).
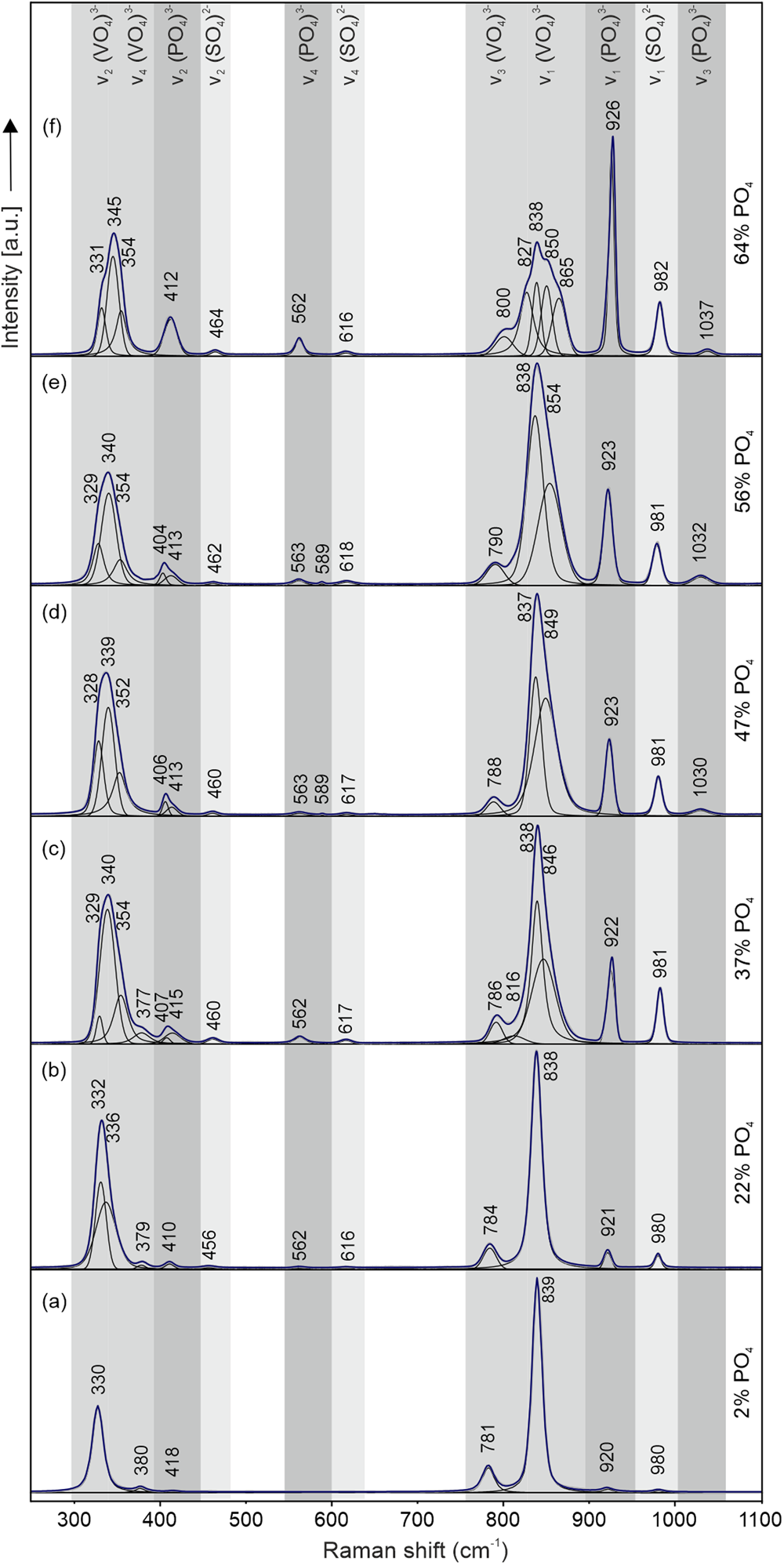
Figure 5. Position of the (PO4)3–, (VO4)3– and (SO4)2– vibrational modes in Raman spectra of the mazorite–gurimite solid-solution series: (a) Raman spectrum of holotype gurimite from Israel; (b–f) Raman spectra of the mazorite–gurimite solid-solution from the mazorite holotype sample.
The occurrence of Raman shifts assigned to the vibration modes of (VO4)3– tetrahedra is more complex. The spectra in Fig. 5 show that the single band at ~838 cm–1 related to the ν1 modes of (VO4)3– retains its position. However, it changes significantly due to increased PO4 content >30%. Modifications such as change of full width at half-maximum (FWHM), broadening, and the appearance of additional components are observed for these vibrational modes (Fig. 5c–f). In turn, the band corresponding to the ν3 modes of (VO4)3– at 781 cm–1 for holotype gurimite (Fig. 5a) moves toward the higher wavenumbers by 19 cm–1 and is placed at ~800 cm–1 for the mazorite with 64% PO4 (Fig. 5f). Raman bands related to the symmetric bending ν2 modes, similar to the ν1 modes of (VO4)3–, are due to broadening and are split into several components due to the increase in PO4 content. The wavenumber of the main band component changes from 330 cm–1 (Fig. 5a) to 345 cm–1 (Fig. 5f). The bands attributed to the asymmetric bending (ν4) modes indicated a slight band shift of ~3 cm–1 and are absent when the proportion of mazorite end-member increases (Fig. 5a–c).
The presence of bands assigned to the sulfate vibrations is related to their contribution to the chemical composition of the mazorite–gurimite series. The content of SO4 in the presented spectra varies between 0.10 to 0.30 apfu, which also causes a slight band movement. The bands attributed to the symmetric stretching ν1 modes of (SO4)2– moved towards higher wavenumbers from 980 cm–1 (Fig. 5a) to 982 cm–1 (Fig. 5f). The Raman shift assigned to the symmetric bending ν2 modes is ~8 cm–1: from 456 cm–1 for gurimite with 22% PO4 to the 464 cm–1 for mazorite with 64% PO4 (Fig. 5b, f), while the bands correlated with the ν4 modes remain almost unchanged at ~617 cm–1.
The frequency of vibrational modes in the mazorite–gurimite series shifted to higher wavenumbers when the contribution of phosphate anion increased. This is connected to the increase in bond strength in the molecule, which depends on the differences in the ionic radius, the atomic mass, and the bond length between the substituted ions (Solecka et al., Reference Solecka, Bajda, Topolska, Zelek-Pogudz and Manecki2018). The prevalence of smaller and lighter P5+ over much larger V5+ in the same tetrahedral site leads to progressive contraction of unit-cell volume. In turn, complex and characteristic modifications of the vibration modes assigned to the vanadate group may be caused by the larger mass of the central atom or by the reduction of the symmetry within the analysed molecule. This can also be correlated with changes in the vibrational dynamics of both cations at one crystallographic site (Juroszek et al., Reference Juroszek, Krüger, Banasik, Vapnik and Galuskina2018a; Solecka et al., Reference Solecka, Bajda, Topolska, Zelek-Pogudz and Manecki2018)
Crystal structure of mazorite and gurimite
The crystal structure of mazorite with its characteristic sheet arrangement perpendicular to c is presented in Fig. 6. In this structure, both symmetrically non-equivalent M sites are occupied by Ba atoms (Fig. 6a) and partially substituted by K (Table 2). The population refinement of these sites converges at 0.937(17) Ba1 and 0.063(17) K1 on the M1 and 0.811(15) Ba2 and 0.189(15) K2 on the M2 site, respectively. The M1O12 polyhedra are linked by edges to each other and form layers perpendicular to c (Fig. 6a). Twelve oxygen atoms coordinate M1, with six longer M1–O1 = 3.2688(8) Å and six shorter M1–O2 = 2.742(3) Å bonds. In turn, the M2O10 polyhedra share square faces and corners and form sheets also perpendicular to c (Fig. 6a). The ten-fold coordinated M2 site is linked to one O1 with a bond length equal to 2.660(5) and nine O2 atoms with relatively longer bonds of 2.893(3) to 2.894(2) Å. The P atoms on the T site in the mazorite structure are tetrahedrally coordinated, with one shorter bond of 1.580(6) Å to the apical O1 atom and three longer bonds of 1.589(2) Å to O2 atoms in the equatorial plane. The six edges of the tetrahedra are shared with M-polyhedra. Due to the partial substitution of V at the T site (ca. 0.77% P and 0.23% V), tetrahedra display slightly longer bond lengths (average P–O = 1.587 Å) compared to pure PO4 groups, with the average P–O = 1.547 Å reported by Sugiyama and Tokonami (Reference Sugiyama and Tokonami1990). Along the c-axis, columnar arrangement characteristic for palmierite-supergroup minerals is also observed in the mazorite crystal structure showing the following order TO4–M2O10–M1O12–M2O10–TO4 (Fig. 6b).

Figure 6. (a) The structure of mazorite as a three-dimensional anionic framework constructed of M1O12 and M2O10 polyhedra, and TO4 tetrahedra, where M = Ba2+ and K+, and T = P5+, V5+ and S6+. (b) The sequence of polyhedra TO4–M2O10–M1O12–M2O10–TO4 along the c-axis in the mazorite structure – characteristic for minerals of the palmierite supergroup.
Because mazorite occurs only in tiny amounts, attempts to collect powder XRD data were unsuccessful. Consequently, the powder XRD pattern was calculated with the program Jana2006 (Petříček et al., Reference Petříček, Dušek and Palatinus2014) using the results of the single-crystal structure refinement. The diffraction lines (I >2%) are listed in Supplementary Table S1.
Gurimite was also analysed using SC-XRD due to the size and good quality of the crystals, for comparison as the vanadium analogue of mazorite (Table 3, sample AM2, note highly enriched in P5+), and as previously only electron back-scatter diffraction (EBSD) data have been available (Galuskina et al., Reference Galuskina, Galuskin, Vapnik, Prusik, Stasiak, Dzierżanowski and Murashko2017). The crystal structure was refined in space group R $\bar{3}$m with the following unit-cell parameters: a = 5.7186(10) Å, c = 21. 2436(4) Å and V = 601.642(19) Å3, which agrees well with previously reported EBSD data. The crystal structure of P-rich gurimite exhibits the layered-type structure made of Ba1O12 and Ba2O10 polyhedra and VO4 tetrahedra. Similarly to mazorite, both Ba layers are connected to each other via face sharing. The partial substitution of V5+ by P5+ affects the reduction of bond lengths in the tetrahedra. In the P-rich gurimite structure, the average length of the V–O bond is equal to 1.6447 Å, whereas for pure Ba3(VO4)2, this value is ~1.7097 Å (Mugavero et al., Reference Mugavero, Bharathy, McAlum and zur Loye2008). More details on the gurimite structure are available in Table 1 and the cif in the Supplementary Materials.
Discussion
Mazorite and its VO4-analogue gurimite are found in the accessory constituents of gehlenite–rankinite paralava, in areas of unusual Ba mineralisation, concentrated in small enclaves in rankinite crystals or interstices of paralava rock-forming minerals. This specific formation has been described as an effect of residual melt crystallisation (Galuskina et al., Reference Galuskina, Galuskin, Vapnik, Prusik, Stasiak, Dzierżanowski and Murashko2017; Krzątała et al., Reference Krzątała, Krüger, Galuskina, Vapnik and Galuskin2020). In other words, the fast crystallisation of the paralava rock-forming minerals leads to the formation of small portions of residual melt, enriched in Ba, K, Nb, Ti, P, V, U, S, F and other incompatible elements, which are the source for rare Ba-bearing minerals. Generally, the mineral composition of the paralava indicates that they could have developed under high-temperature conditions above 1000°C, with low pressure and high oxidising potential (Vapnik et al., Reference Vapnik, Sharygin, Sokol and Shagam2007; Sharygin et al., Reference Sharygin, Sokol and Vapnik2008; Krzątała et al., Reference Krzątała, Krüger, Galuskina, Vapnik and Galuskin2020). Furthermore, the synthetic Ba3(PO4)2 and Sr3(PO4)2 were successfully synthesised at a high-temperature above 1000°C (Sugiyama and Tokonami, Reference Sugiyama and Tokonami1990), confirming that mazorite, as well as gurimite, can indeed form as a high-temperature mineral. The formation of the mazorite–gurimite solid-solution crystals found in gehlenite–rankinite paralava is most probably connected with a similar crystallisation mechanism.
It is well-known that continuous solid-solution can occur when ions of one type substitute for ions of another type in a structure, forming a single crystalline phase. According to Goldschmidt's rule, complete isomorphism is only possible between ions whose ionic radii differ by no more than 10–15% (Goldschmidt, Reference Goldschmidt1926). The difference between ionic radii of P5+ (0.17 Å) and V5+ (0.355 Å) (Shannon, Reference Shannon1976) is greater than 50%. However, substitutions between (PO4)3– and (VO4)3– ions are not uncommon, and a few mineral solid-solutions exist. A well-known solid solution is between pyromorphite Pb5(PO4)3Cl and vanadinite Pb5(VO4)3Cl (Baker, Reference Baker1966; Solecka et al., Reference Solecka, Bajda, Topolska, Zelek-Pogudz and Manecki2018) or between the tellurates: kuksite Pb3Zn3(TeO6)(PO4)2 and cheremnykhite Pb3Zn3(TeO6)(VO4)2 from the Kuranakh gold deposit (Central Aldan, southern Yakutia) (Kim et al., Reference Kim, Zayakina and Makhotko1990). Furthermore, in the pyrometamorphic rocks of the Hatrurim Complex, two solid-solutions involving (PO4)3– ↔ (VO4)3– substitution have been reported. The first is between zadovite BaCa6[(SiO4)(PO4)](PO4)2F and aradite BaCa6[(SiO4)(VO4)](VO4)2F (Galuskin et al., Reference Galuskin, Gfeller, Galuskina, Pakhomova, Armbruster, Vapnik, Włodyka, Dzierżanowski and Murashko2015), and the second one described recently, between fluorapatite Ca5(PO4)3F and pliniusite Ca5(VO4)3F (Pekov et al., Reference Pekov, Koshlyakova, Zubkova, Krzątała, Belakovskiy, Galuskina, Galuskin, Britvin, Sidorov, Vapnik and Pushcharovsky2022). However, there are significant differences between these mineral series. In the case of the zadovite–aradite solution, primary investigations confirm the occurrence of continuous series (Galuskin et al., Reference Galuskin, Gfeller, Galuskina, Pakhomova, Armbruster, Vapnik, Włodyka, Dzierżanowski and Murashko2015), similar to the mazorite–gurimite solid solution presented in this work, although samples with a composition close to the Ba3(PO4)2 and Ba3(VO4)2 end-members were not found. In addition similar formation conditions are characteristic for both the mazorite–gurimite and the zadovite–aradite mineral series. They have crystallised from the residual melt within the small enclaves of gehlenite–rankinite paralava at a very high temperature of ~1000°C. In contrast, a solid solution between fluorapatite and pliniusite is discontinuous, with a characteristic gap between both end-members. In this case, fluorapatite crystallised at the early stage together with the rock-forming minerals of paralava. In turn, pliniusite, similar to other accessory Ba-bearing minerals mentioned above, forms later from residual melt enriched in vanadium (Pekov et al., Reference Pekov, Koshlyakova, Zubkova, Krzątała, Belakovskiy, Galuskina, Galuskin, Britvin, Sidorov, Vapnik and Pushcharovsky2022). Regardless of the formation temperature, the appearance of a continuous solid solution is mainly related to similar melt chemistry, which allows for a complete isomorphism.
In Table 5, the structural parameters obtained from the SC-XRD data for mazorite (Ba2.56K0.44)(P1.54V0.46)O8 and P-rich gurimite (Ba2.58K0.42)(V1.04P0.96)O8 are compared with the synthetic end-members Ba3(PO4)2 (Sugiyama and Tokonami, Reference Sugiyama and Tokonami1990) and Ba3(VO4)2 (Mugavero et al., Reference Mugavero, Bharathy, McAlum and zur Loye2008). The changes in chemical composition, especially (VO4)3– ↔ (PO4)3– substitution, have major effects on the unit-cell volume, which decreases from Ba3(VO4)2 to Ba3(PO4)2. The same effect is visible in gradual changes of the bond lengths in tetrahedra, decreasing from 1.710 Å in pure VO4 (Mugavero et al., Reference Mugavero, Bharathy, McAlum and zur Loye2008), to 1.654 Å in P-rich gurimite (V0.52P0.48), to 1.587 Å in mazorite (P0.77V0.23), and 1.547 Å in pure PO4 (Sugiyama and Tokonami, Reference Sugiyama and Tokonami1990). Cation substitution can affect the distortion of the polyhedra in the structure. However, for the phases presented in Table 5, the calculated quadratic elongation parameter (λ) (Momma and Izumi, Reference Momma and Izumi2011) does not indicate a significant distortion of tetrahedra because the slight deviation from regular polyhedron (λ = 1) is assigned only to V-rich members.
Table 5. Correlation of structural parameters for synthetic and natural members of the mazorite–gurimite series.

Identifying and describing the new mineral mazorite, as in any unique and rare phase case, is key to understanding the complexity of diverse mineralogical environments and may help resolve some genetic aspects of high-temperature rocks. Furthermore, its identification allows us to affirm the presence of a continuous solid solution between mazorite and its vanadate analogue, gurimite (Galuskina et al., Reference Galuskina, Galuskin, Vapnik, Prusik, Stasiak, Dzierżanowski and Murashko2017). In addition, the chemical data presented in this work (Table 2) confirm the possibility of finding new structurally similar counterparts, such as a molybdenite analogue of gurimite.
Acknowledgements
The authors thank Bernd Ternes from Mayen, Germany, for collecting carbonate–silicate xenolith samples from the Bellerberg volcano, in which mazorite was also detected. H.K. and V.K. acknowledge the help of Francesco Pagliaro during the data collection at X06DA. Vincent Olieric is thanked for setting up the beamline and donating extra beamtime. The authors are also thankful to Sergey Aksenov, Peter Leverett and Igor Pekov for their helpful and constructive comments, which improved the manuscript. The investigation was partially supported by the National Science Center of Poland Grant (grant number 2021/41/B/ST10/00130).
Supplementary material
The supplementary material for this article can be found at https://doi.org/10.1180/mgm.2023.57.
Competing interests
The authors declare none.