1.1 Overview
1.1.1 Introduction
In this chapter, the subject of the book is introduced to the reader. Classifications of different types of turbomachine and different types of compressor are reviewed, to define precisely what is meant by a radial flow turbocompressor. This first chapter also considers the basic operating principle of turbocompressors, known as the Euler turbine equation, which is valid for all turbomachines. The function of the components of a single-stage compressor are introduced, and the many different forms of single-stage and multistage compressors are described. An overview of different types of impeller is provided.
Centrifugal compressors have become ubiquitous over the twentieth century across a wide range of applications due to their inherent robustness, good efficiency and broad operating range. Numerous applications of radial flow turbocompressors are described in this chapter whereby the special aerodynamic features that are relevant to their design for each application are highlighted. The wide range of applications explains why the book is devoted to this relatively narrow part of the wide field of turbomachines. Even small gains of efficiency, improvements in operating range and an increase in swallowing capacity are welcomed across all industrial applications.
Some comments are provided in the chapter on the effect of renewable energy on the applications of radial compressors. The future of centrifugal compressors seems equally bright with the present focus on environmental goals, including the future decarbonisation of power generation and transportation, with a possible shift to a hydrogen economy. There is ever-increasing pressure to produce higher-efficiency gas turbines at increased cycle pressure ratios for aviation propulsion and ground-based power generation, which also favour the use of centrifugal or axial-centrifugal machines.
Aspects of the history of turbomachines are also given together with a short overview of some other useful books on this topic.
1.1.2 Learning Objectives
Define what is meant by a turbomachine.
How does a turbocompressor differ from other rotary compressors?
Define the differences between turbines and compressors, axial and radial machines and thermal and hydraulic turbomachines.
Know the basic operating principle of a turbomachine based on the Euler turbine equation.
Be able to identify various applications of radial flow turbocompressors.
Have a broad knowledge of the history of turbomachinery with emphasis on radial flow turbocompressors.
Be aware of other useful books on the same subject matter.
1.2 Definition of Turbomachinery
Turbomachines are rotating machines used to change the state of a working fluid – liquids in pumps, gases in compressors and one or the other in turbines. Pumps, fans, ventilators and blowers are used to transport fluids; turbines and wind turbines extract energy from a fluid stream. Turbochargers, propellers and jet engines constitute part of propulsion or transportation devices. This wide remit means that turbomachines span almost all industrial sectors and play a vital role in many of them. They are often only a small part of a more complex system, which typically imposes the requirements for and constraints on their design. There is a huge range of types, sizes and speeds, with large economic significance in numerous applications. In addition, the technological and scientific interest of fluid dynamics, thermodynamics and mechanics makes turbomachinery a highly worthwhile subject of engineering study.
Turbomachinery is fundamentally linked to energy conversion in its many forms. Most electrical power is currently generated by steam turbines in nuclear and coal-fired plants, but even modern solar or biomass power plants use small steam or gas turbines for energy conversion. Natural gas is the fuel most commonly used for land-based gas turbine power plants, and nowadays these are often combined with a steam turbine which, in turn, obtains its heat source from the hot exhaust gases of the gas turbine. Water turbines in hydroelectric schemes and wind turbines use renewable energy sources for power generation.
Turbomachinery plays an equally important role in the transportation industry – the gas turbine jet engine is used for propulsion in nearly all aircraft; turbochargers are widely used in ground transportation and as an integral part of diesel propulsion in nearly all ships, lorries and diesel cars and, increasingly, in gasoline-fuelled vehicles. Pumps are the world’s most ubiquitous turbomachines, found everywhere where liquid needs to be transported. Compressors and pumps are important to chemical processes, to the use of industrial gases and in the oil and gas industries. Compressors and ventilators are key components in air conditioning, cooling and refrigeration equipment. Turbomachinery can even be found in the medical industry, where pumps are deployed for blood circulation, ventilation-assist fans are used in the treatment of sleep apnoea and in other clinical applications. The drive powering a high-speed dental drill is, in fact, generally a tiny high-speed air-driven turbine.
The distinguishing feature of a turbomachine is that energy is transferred continuously to or from a fluid by the aerodynamic action of the flow around rotating blades in an open system. Different aspects of this are discussed later in this chapter.
1.2.1 Open System
The definition of an open system is discussed in Chapter 2 on the thermodynamics of energy transfer. To explain a turbomachine as part of an open system, it is useful to make a simple comparison between the work-producing processes in a gas turbine and those of an internal combustion engine. In the gas turbine, the energy transfer takes place through the continuous aerodynamic forces of the gas passing through the flow channels of a machine which is open at both ends. There are no valves or movable plates to force the flow to pass forwards through the machine or to stop the flow from reversing direction, as is the case in a reciprocating machine.
The different parts of the gas turbine process take place continuously in separate components which are open at both ends and are specifically designed to achieve high efficiency for each purpose: the intake, the compressor, the combustion chamber and the turbine and, in a jet engine, the outlet nozzle. Work is produced in the rotor blade rows of the turbine, and this is used as motive power to drive the compressor. The excess power not needed by the compressor is then available to drive a generator or to provide thrust for an aero engine.
In the internal combustion engine, similar processes take place but the energy is transferred by the intermittent operation of forces acting on the pistons in a closed cylinder, which are forced to change direction regularly. There are valves which open and close to trap gas in the cylinder and to allow it to enter and leave during the different expansion and compression processes. These variable processes take place in the same component but at different times, and the work transfer is intermittent. In a two-stroke engine, there is one power stroke every two strokes of the piston in a single revolution of a shaft; in a four-stroke engine, two shaft revolutions are needed for each power stroke.
In a gas turbine, there are no strokes, since each blade passage provides more or less continuous flow and ensures that the forces acting on the shaft, with the exception of the small variations due to the interaction of the moving and stationary blade rows, are steady. Because of this, a turbomachine basically has only one moving part. This rotates rather than oscillates, and has high reliability and a long lifetime. The continuous rotating motion applies relatively simple mechanical loads, and the rotors may achieve high rotational speeds, resulting in high power density – turbomachines that are generally light in weight for a given power.
1.2.2 Continuous Energy Transfer by Flow over Blades Rotating around an Axis
Turbomachines differ from positive displacement devices in that they effect a continuous energy transfer. In the case of positive displacement machines, the work is transferred discontinuously by changing the volume of a trapped mass of fluid, releasing it and then intermittently repeating the process. Most positive displacement machines are reciprocating – as in a piston engine – but some, such as screw compressors, liquid ring compressors and sliding vane blowers, may have rotary motion and be nearly continuous in operation.
The limitations of the intermittent power and mass flow capability associated with reciprocating machinery explains the fact that the largest diesel engine (for container ships) has a power output of about 80 MW, compared with typically 1600 MW for the largest single-shaft steam turbines. In contrast to discontinuous operation, a large energy flux can be obtained in turbomachines and hence high gas flow rates require only comparatively small components. In addition, rotary motion is a natural characteristic of turbomachinery and does not require complex oscillating connecting rods from the machine crankshaft to a rotating shaft.
A categorisation of different compressor types is given in Figure 1.1. The piston compressor comprises a reciprocating piston and associated valves and operates on the same principle as a bicycle pump. A diaphragm compressor comprises a flexible diaphragm which is moved by means of a hydraulic system. The movement of the diaphragm draws the process gas into a chamber enclosed by the diaphragm and then forces it under pressure through a valve. Another possibility, often used for vacuum applications, is to use a jet of gas at high pressure in an ejector to transfer its momentum to a stream of gas at low pressure.

Figure 1.1 Categories of different compressor types.
Screw compressors use two helical screws, both known as rotors, which mesh to enclose a small volume of gas. Gas enters at the suction end of the casing and moves through the threads of the screws as they rotate and is then forced at pressure out of the exit of the compressor casing. The rotary lobe compressor also consists of two rotors that have two or three lobes. The rotors rotate such that air is sucked into the inlet and is forced out of the discharge by the lobes. The liquid-ring compressor has a vaned impeller rotating eccentrically within a cylindrical casing. Liquid (usually water) within the casing is centrifuged outwards and provides a liquid ring that seals the space between the impeller blades and the casing. The eccentric impeller results in a periodic variation of the volume enclosed by the vanes and the liquid ring; this draws air into the casing and delivers it at a higher pressure. A sliding vane compressor also has an eccentric rotor, but the blades themselves are able to move within the rotor to seal the casing. The blades are held in close contact with the outer shell under the action of the centrifugal force, and the sealing between the vanes and the casing is improved by the injection of a lubricating oil over the entire length of the blade. The main drawback of positive displacement compressors is that the compression cycle is discrete: the gas comes in bursts rather than smoothly and continuously.
On a timescale of the order of seconds, the time-averaged properties within a turbomachine can be considered as a smooth and continuous steady flow process, at least at the usual stable operating points. However, the forces acting on a blade are not steady at very small timescales (milliseconds). The aerodynamic interaction of the motion of adjacent blade rows leads to small, unsteady variations in pressure at frequency with which the blades and vanes pass each other. In fact, these variations can force blade vibrations, and if they are not under control the mechanical damage can severely interfere with the life and aerodynamic function of the machine. In fact, a classical analysis by Dean (Reference Dean1959), clarified by Hodson et al. (Reference Hodson, Hynes, Greitzer and Tan2012), shows that the equations describing the change in enthalpy for a fluid particle in a turbomachine require a time variation in pressure. Conveniently, for most analyses the design engineer is concerned with the mean, time-averaged performance and may consider the smooth operation to be steady.
1.2.3 Aerodynamic
The truly key feature of a turbomachine is the aerodynamic forces acting on the blades. It is the motion of the fluid compared to the motion of the machine that is primary. The actual motion of the fluid in a positive displacement machine is, by contrast, generally less well ordered and is secondary to the motion of the components – such as pistons or valves. As the flow passes over the blade surface of a turbomachine, an aerodynamic lift force is generated, similar to the lift force on an aircraft wing. If the blades are moving in the direction of this force, then there is a transfer of work between the fluid and the shaft. This is the essence of turbomachinery, and a good understanding of fluid dynamics is therefore one of the key building blocks in the design of good turbomachines.
As an example of the importance of aerodynamic forces, it is useful to consider the work input into an Archimedean screw. This rotating machine acts as a pump to raise water to a higher level (or as a water turbine when the water is flowing downhill), but it is not a turbomachine because the work input is not related to an aerodynamic force. Instead, it is due to work done against gravitation to lift the weight of the water as it periodically fills and empties from a section of the screw.
Similarly, one can distinguish between undershot and overshot water wheels. In an overshot water wheel, the water enters at the top of the wheel and the weight of the water held in each bucket provides the necessary torque. This is not a turbomachine as long as the small impulse from the water entering and leaving the buckets can be neglected. An undershot wheel, by contrast, makes use of the kinetic motion of the fluid to provide a force on the paddle blades. The head of water must first be converted into kinetic energy or the wheel needs to be mounted in a fast-running stream. The undershot water wheel is considered to be a turbomachine as its operation is due to an aerodynamic force from the motion of the water acting on the blades. In this case, however, it is a drag force and not an aerodynamic lift force, which is more normal in turbomachines.
The range of types and sizes of turbomachines is vast. Common examples in rough order of increasing size are: high-speed dental drills, computer cooling fans, hair dryers, ventilation fans, turbochargers for cars, water pumps, blowers for air compression, compressors and turbines for helicopter engines, turbochargers for ships’ diesel engines, turbines and compressors in aero engines and industrial gas turbines, water turbines, steam turbines and wind turbines in the power generation industry. If world records are considered, wind turbines are the largest (having a rotor tip diameter above 200 m), Francis turbines are the most powerful in terms of the energy from a single blade row (with a power of 800 MW from a single impeller with a diameter of between 5 and 10 m), and the steam turbine is the most powerful turbomachine on a single shaft.
Practical radial turbocompressors can be found in a range of applications with impeller diameters, D2, of between 10 mm and 2000 mm, and an impeller tip-speed, u2, of between 100 and 700 m/s. An overview is provided in Figure 1.2. Low-pressure compressors with low tip-speeds are known as fans and medium-pressure compressors as blowers. While most of the fundamental principles of design can be comfortably applied across the range of size and tip-speed, some aspects do not scale. For example, the tip clearance of open impellers is one of the key factors limiting the efficiency of small stages, and the blade thickness tends to become larger relative to the diameter at small size due to manufacturing constraints. In many applications at the larger sizes, it may be more efficient – and may reduce the outer dimensions – to use a smaller axial compressor or an axial-centrifugal compressor. Large centrifugal machines are often preferred, however, as they are more robust and have much wider operating characteristics.
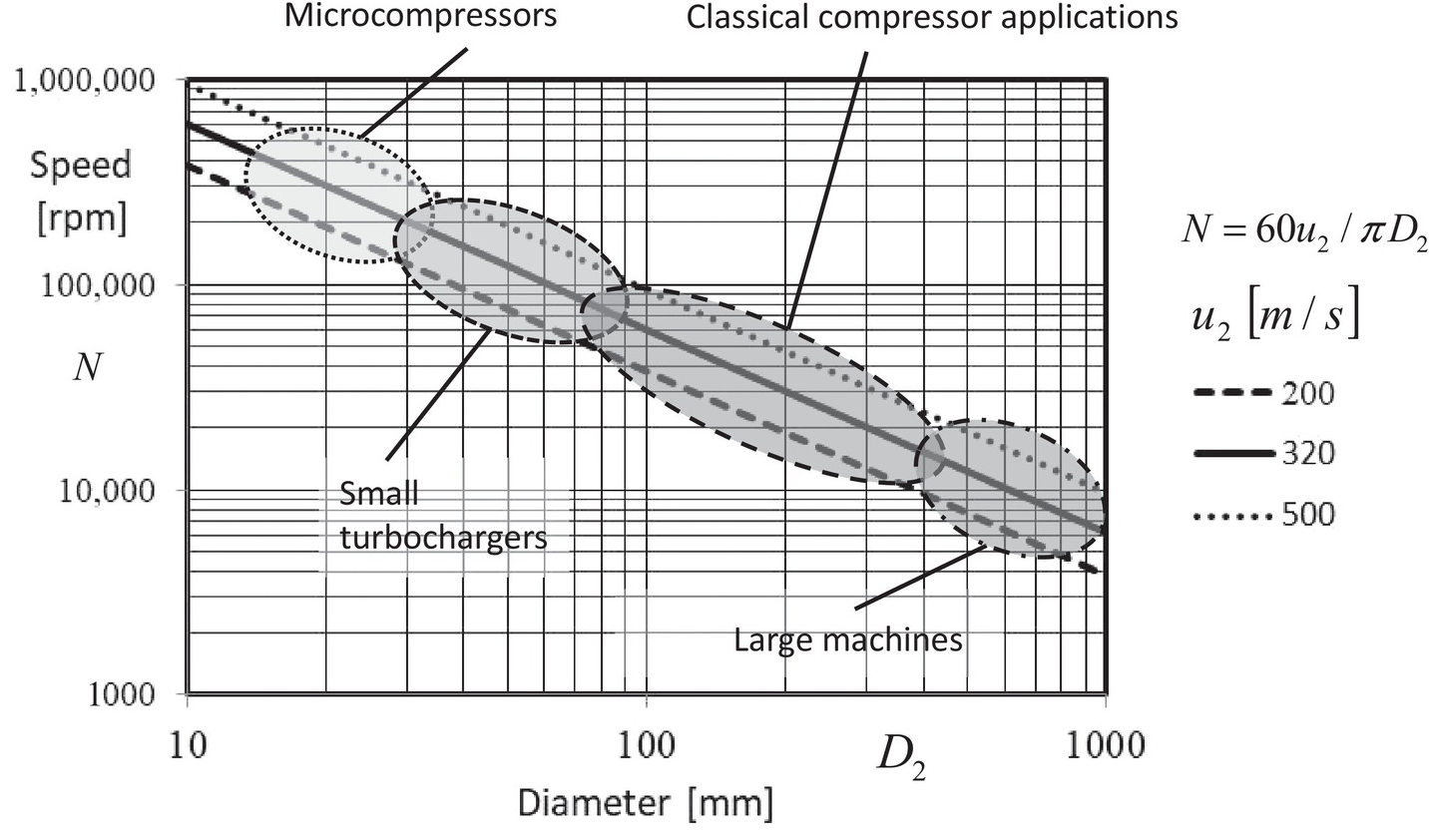
Figure 1.2 An overview of the range of size and rotational speed of radial flow turbocompressors.
1.2.4 Principle of Operation
An understanding of the fact that machines of such a broad range of power density and application operate on the same single physical principle is the key to effective turbomachinery design. The unifying theoretical system is the Euler turbine equation, first derived from Newton’s second law by the polymath Leonhard Euler (1707–1783) in the eighteenth century.
Newton showed that a force is needed to generate a change in the speed of an object, and the change in speed is proportional to, and along the direction of, the applied force. Euler extended this and deduced that the torque acting on a turbomachinery shaft is related to the forces causing a change in the angular momentum of the gas by a change in its circumferential velocity between the inlet and outlet of a blade row. This is illustrated in Section 2.2 and discussed in detail in most other chapters. When the Euler turbine equation is applied to the gas passing from the inlet (station 1) to outlet (station 2) across a rotor blade row, it gives the specific shaft work (the mechanical work per unit mass flow on the shaft) in terms of the changes in the circumferential component of the gas velocity (cu) and the blade speed (u) as
The individual blade rows guide the fluid as it passes through the flow channels and produce a change in direction of the swirling flow relative to the circumferential direction. It is this change in angular momentum across a rotor which creates work on the shaft: such that the principle of a turbomachine can be thought of as a machine in which the blades cause a change in the swirl of the flow. The addition of swirl in a rotor blade row of a compressor is usually followed by its removal in a downstream stator row, and so a turbomachine usually has at least two blade rows.
Not only is the Euler turbomachinery equation universally applicable, it also combines great simplicity and elegance. Furthermore, it is formidably potent: as it is based on a control volume approach, it determines the work of the machine simply from the changes between the mean conditions at inlet and outlet of a rotor with no knowledge of the inner workings of the blade row. It is immediately clear from the dimensions of this equation, for example, that the work done per unit mass on the fluid in a compressor is proportional to the product of two velocities and thus to the square of the blade speed. Therefore, for compressors with high pressure ratios, high blade tip-speeds are required.
In all turbomachines, at least one blade row rotates, which means that a fundamental aspect of the application of the Euler turbomachinery equation is the consideration of the flow in the blade rows, both in an inertial system relative to the moving blades and in the absolute frame of reference. The stator vanes guide the direction of the flow in the absolute frame, while the rotor blades guide the relative flow in the rotating coordinate system. An important aspect is then the relationship between flow in the absolute coordinate system, as seen by an observer outside the machine, and the flow in a relative coordinate system that rotates with the rotor. This approach was first formulated in the theory of steam turbines by Aurel Stodola (1859–1942) (Stodola, Reference Stodola1905). The transformation of velocity vectors from one system to the other requires vector addition of velocities. At each point in the flow through a turbomachine, the absolute velocity, c, and the relative velocity, w, can be identified and combined through the local circumferential blade speed, u, with a so-called Galilean transformation between the two inertial systems:

The Galilean transformation of velocities leading to velocity triangles is discussed in Section 2.2.5.
Another simple description of the principle of operation of a compressor is sketched in Figure 1.3, which depicts the change in the nondimensional absolute velocity and static pressure through a 2D compressor stage. The absolute gas velocity, c, increases through the impeller along together with the static pressure. At the impeller outlet, the absolute gas velocity almost reaches the tip-speed of the impeller. The deceleration of the gas in the downstream diffuser leads to a further rise in pressure. The proportion of the pressure rise in the impeller to that in the whole stage, in this case about 60%, is called the degree of reaction.

Figure 1.3 Dimensionless absolute gas velocity and pressure rise in a 2D compressor stage.
The aerodynamic forces and the stresses resulting from high-speed rotation must be safely carried by the blades and by the other mechanical elements. In addition, the blades need to stay attached to the rotor. Special attention must be given to the sealing between the rotating elements and the stationary elements. Some of the unsteady stresses in turbomachinery blade rows are associated with vibration phenomena from blade row interactions and flow instabilities, both of which may lead to material failure by fatigue. Stress analysis, vibration analysis, the selection of materials and the mechanical component design for safe operation are key aspects in the integration of turbomachinery components into actual products and are introduced in Chapter 19 of the book.
1.3 Classification of Turbomachines
1.3.1 Power Consuming and Power Producing Machines
There are many ways to classify turbomachines. This book covers only a fraction of the many categories of turbomachinery, as it deals with thermal and not hydraulic machines, radial and not axial machines, compressors and not turbines, and only includes machines enclosed within a casing, as explained in this section.
The most fundamental classification is into machines which add energy to a fluid and those which extract energy from a fluid: work-absorbing machines and work-producing machines. The addition or extraction of energy is usually achieved at the expense of the fluid pressure. Turbines have an output of shaft work from the machine which they obtain by converting the internal energy of the fluid into rotational energy of the shaft. In all turbines, except wind turbines, the pressure falls as rotational kinetic energy is generated in upstream components, and this energy is then extracted by the rotor blades. Compressors (and pumps) require work input to the shaft to generate a pressure (or head) rise. The work is first converted into static pressure or enthalpy rise and an increase in kinetic energy in a rotor blade row. The kinetic energy is then converted into a further pressure rise by a stator blade row. In some low-cost applications, such as ventilator fans, the downstream stator is not present and the exit kinetic energy is simply discarded.
1.3.2 Thermal and Hydraulic Machines
The distinguishing feature of a thermal turbomachine, as opposed to a hydraulic turbomachine, is that the working fluid is compressible. The gas undergoes a marked change in volume with the density and temperature changes that occur as it passes through the machine. In a hydraulic machine, the fluid (usually cold water) is effectively incompressible and the density remains constant: the fluid then has the same volume, and very nearly the same temperature, at the inlet and the outlet of the machine. Thermodynamics is not relevant to the performance analysis in this case.
A multistage pump has similar impellers in all of its stages as there is no difference in the volume flow at different positions in the machine. On the other hand, a multistage compressor requires that the flow channel of the stages is adapted to account for the decrease in volume of the gas being compressed, which means that different impellers with narrower flow channels are needed on the passage through the machine. Figure 1.4 from Neverov and Liubimov (Reference Neverov and Liubimov2018) shows this for a multistage machine with three different open impellers of the same diameter on the same shaft. The amount of volume compression for a given pressure rise is also a function of the gas properties of the fluid being compressed so that different impellers are also needed for different gases. The manufacturer of multistage pumps needs to develop a single stage that can be used at each position in a multistage machine as the volume flow does not change through the machine, whereas the compressor manufacturer needs to develop or adapt separate stages for each location.

Figure 1.4 The shaft of a three-stage air compressor with open impellers manufactured by Entenmach RPC LCC, Saint Petersburg, Russia.
A limiting case in respect of thermal turbomachinery is a low-speed ventilator where the density change is almost negligible and so these machines may be regarded as incompressible. The large changes in temperature and density in high-speed turbomachines can only be properly accounted for by the theory of compressible gas dynamics. This is another key building block to understanding the design and operation of turbocompressors and is covered in Chapter 6 of the book.
1.3.3 Acceleration and Deceleration of the Flow
The addition or extraction of energy is usually made at the expense of the fluid pressure; compressors generate a pressure rise, whereas turbines cause a pressure drop. Furthermore, whether the static pressure rises or falls through the machine is an important difference. In compressors and ventilators, the static pressure usually rises in the direction of flow, whereas it usually drops in the direction of flow in turbines. It is fundamentally difficult to persuade a fluid to move ’uphill’ against rising pressure. This means that the basic aerodynamic design of compressors is generally more problematic and more affected by aerodynamic limits than that of turbines.
Compressor blade rows generally experience decelerating flow as the flow gives up its kinetic energy to produce a static pressure rise. This tends to cause the slow-moving fluid in the thin fluid boundary layers on the blades to become slower and, as a consequence, the boundary layers become thicker and are more liable to separate from the blade surface. The propensity for flow to separate means that the rate of reduction in velocity or the rise in pressure must be carefully managed, and this effectively results in a limit on the deceleration of the flow for compressor stages. Turbines, with accelerating flow in the direction of the pressure gradient, tend to have thin, stable boundary layers on the blades, and there is no limit to the amount of acceleration that the fluid encounters.
This fundamental difference between the two types of machine is evident from the cross section of any industrial single-shaft gas turbine – where there are usually three to four times as many compressor stages as turbine stages, although both have the same rotational speed and nearly the same pressure ratio.
Some turbomachines, such as a reversible pump-turbine for a pumped hydroelectric storage scheme, operate as both turbines and compressors. At times of low electrical demand, excess generation capacity is used to pump water into the higher reservoir. When there is higher demand, water is released into the lower reservoir through the same machine, which is now operating as a turbine and generating electricity. The design for pump operation takes priority; the machine will operate adequately as a turbine with accelerated flow when the water runs downhill, albeit nonoptimally.
1.3.4 Flow Direction
Another important criterion for the classification of turbomachinery is the flow direction relative to the axis of rotation. In purely axial rotors, the radius of the streamlines is approximately constant and the flow passes through the machine roughly parallel to the rotational axis. In purely radial machines, the radius changes significantly and the flow travels through the rotor perpendicular to the axis of rotation, as shown in Figure 1.5. In practice, axial machines tend to have some small radial velocity components as neither the inner nor the outer casing walls are perfectly cylindrical, and most radial machines have an axial component of velocity. Most radial flow compressors have axial flow at the inlet and are radial at the outlet (and vice versa for radial flow turbines), as shown in Figure 1.5.

Figure 1.5 Sketches of axial, diagonal, mixed and radial flow compressors.
In some situations, these may qualify as mixed-flow machines because of the axial inlet and radial outlet. In the present text, the adjectives radial and centrifugal are used for all machines with a significant change of radius across the impeller and with a radial flow at exit. The name centrifugal impeller implies that the effect of centrifugal force (radius change) in generating a pressure rise across the impeller is significant.
Defining the machine type based on the orientation of the leading and trailing edges can become confusing: for an axial inlet flow and radial outlet flow, the leading and trailing edges can also be designed with different sweeps with respect to the mean meridional flow. The term diagonal is sometimes used for rotors which have leading and trailing edges which are neither axial nor radial and are swept forwards or backwards in the flow channel. The term mixed flow can be linked to the direction of the mean flow at the impeller outlet, which then has a large axial component of velocity. This naming convention is mainly relevant to the impeller and not to the entire machine, as turning the meridional flow downstream of the rotor to the axial direction does not change the type of the machine.
In this book, the term meridional direction is often used. This refers to the mean direction of the flow in a meridional plane through the axis of the rotor and can be axial, radial or something in between. This formulation has the advantage that it allows the same equations to be used for the analysis of axial and radial machines; see the notation in Figure 1.6.

Figure 1.6 Axial, radial, circumferential and meridional velocity components.
The key significance of the distinction between axial and radial flow arises owing to the centrifugal effect whereby an object travelling in a circle behaves as if it is experiencing an outward force. In an axial machine with swirling flow, this causes the static pressure to rise towards the casing to counteract the swirling motion of the fluid such that the pressure at the casing is higher than at the hub. This radial pressure gradient causes a natural pressure rise on a swirling fluid particle whenever its radius increases within a rotating blade row, and this pressure gradient accounts for 75% of the static pressure rise in centrifugal compressor impellers. This is sometimes considered to be a free pressure rise as it does not require flow deceleration and does not, in itself, result in any flow separations. The centrifugal effect makes centrifugal stages somewhat easier to design than axial stages for a given pressure rise. For this reason, the first effective gas turbine engines all made use of radial compressors; see Section 1.4.
As shown in Figure 1.3, compressor rotors increase the absolute velocity across the rotor blade rows by adding swirl to the circumferential flow, and they are equipped with a downstream diffuser or row of stator vanes to convert the rotor outlet kinetic energy into a useful pressure rise. Compressor rotors turn the flow from the meridional direction towards the circumferential direction in the direction of rotation. In contrast, turbines are equipped with an upstream stator vane or a casing to provide swirl in the direction of rotation, and this leads to high inlet kinetic energy, which is then extracted by the rotor. The turbine rotor turns the absolute flow velocities from the circumferential direction against the direction of rotation back towards the meridional direction.
1.3.5 Degree of Reaction
The degree of reaction for a turbomachinery stage describes the relative pressure rise (or fall) in the rotor compared to that of the stage. This is often discussed in the context of steam turbines where reaction turbines and impulse designs with no reaction can be found. In the history of steam turbines, both types were invented independently, the reaction turbine by Sir Charles Parsons (1854–1931) and the impulse turbine by Gustaf de Laval (1845–1913). The reaction turbine, as the name implies, is turned by reactive force rather than by a direct push or impulse. An impulse turbine has fixed nozzles (stator blades) that orient the fluid flow into high-speed jets. These jets contain significant kinetic energy, and the rotor blades, shaped symmetrically like buckets, convert this into shaft rotation as the jet changes direction. A pressure drop occurs in the nozzle, but the pressure is the same when the flow enters and leaves the rotor blade, and this gives rise to an impulse machine with zero degree of reaction. In a reaction turbine, the rotor makes use of the reaction force produced as the fluid accelerates through the rotor blades. The fluid is directed on to the rotor by the fixed vanes of the stator. It leaves the stator as a stream that fills the entire circumference of the rotor. In a reaction machine, the pressure change occurs across both the stator and the rotor.
Centrifugal compressors with no inlet swirl are reaction machines and generally have a degree of reaction close to 0.6 indicating that about 60% of the overall static pressure rise of the stage takes place in the rotor, Figure 1.3.
1.3.6 Boundary of the Flow Field
Turbomachines can also be classified with respect to configurations with and without a casing. In machines without a casing, such as windmills, wind turbines, propellers and some axial fans, where no walls exist to support a pressure difference against the ambient pressure, it is essentially the kinetic part of the change in the fluid energy which effects the energy transfer with no change in static pressure.
1.4 Short History of Thermal Turbomachines
1.4.1 The Prefix Turbo
The prefix turbo in the word turbomachine comes from the Latin source – turbo, turbinis, meaning whirlwind, whirlpool, vortex and, by extension, a child’s spinning top. Given that whirlwinds can wreak havoc, a secondary meaning of turbo in Latin was confusion, or disorder, and this can still be identified in English words such as disturb and turbulent. The word turbine was first coined in French in 1828 by Claude Burdin (1790–1873) to describe his submission in a government-sponsored engineering competition for a new water power source. The French authorities of the time were keen on developing water power because they had few natural fossil fuel resources, whereas Britain had abundant energy in the form of coal, which could be used to power steam engines and had made more rapid progress in the early days of industrialisation.
In modern usage, turbo is a prefix for many types of turbomachine, such as turbopump, turbojet, turbofan, turboprop, turboshaft, turbocompressor and turbocharger. A turbopump often refers to a rocket fuel pump. Turbojet, turbofan, turboprop and turboshaft are different architectures of gas turbine aeroengines. Turbochargers are used in internal combustion engines to increase their power by forcing more air into the charge of a cylinder, on the principle that introducing more oxygen means that more fuel can be burned. The use of turbochargers in fast sports cars caused the word turbo to become synonymous with anything superlative and fast. Turbomachinery has a long history and can be considered to be a very mature technology. Despite this, new developments and applications continually take place by applying incremental pieces of knowledge discovered by the many researchers, engineers and companies in the field.
1.4.2 Historical Overview
Examples of turbines can be found in antique literature, such as Hero’s turbine (or aeliopile) as designed by Hero of Alexandria (c. 10–70 AD). The centrifugal pump was invented by Denis Papin (1647–1710) in France at the end of the seventeenth century but was not brought to fruition (Harris, Reference Harris1951). The decisive scientific steps to explain the operation of a pump or a turbine were taken by Leonhard Euler (1707–1783) with the derivation of the Euler turbomachinery equation; see Section 1.2.4. Developments in hydraulic turbomachinery throughout the nineteenth century involving James Francis (1815–1892), Lestor Pelton (1829–1908) and Osborne Reynolds (1842–1912) led to the pioneering work of Sir Charles Algernon Parsons (1854–1931) and to the development in 1884 of the first multistage reaction steam turbine for driving a generator as an electrical power source. Given that about 70% of the world’s power at the end of the last century was still produced in large centralised steam turbine power stations, this is rightly considered to be one of the landmark technological achievements and a watershed leading to today’s energy-intensive society.
John Barber (1734–1793) published the first patent for a gas turbine based on a reciprocating compressor which preceded Parsons’ steam turbine by nearly 100 years, but the development of a practical gas turbine took rather longer. Even the turbocharger is older than the first practical gas turbine, with the first patent being awarded to the Swiss engineer Alfred Büchi (1879–1959) in 1905 for the effective demonstration of the use of radial turbocompressor technology for turbocharging a diesel engine. This became the standard for ship propulsion from around 20 years afterwards. The compressor technology used in his turbocharger was derived from the multistage inline radial compressors patented by Professor Auguste Rateau (1863–1930) in 1899 for providing high-pressure air for blast furnaces in steelworks and for mining ventilation.
The biggest obstacle to the practicable gas turbine at the beginning of its development was the low efficiency of the compressor. An analysis showing the large effect of the compressor efficiency on the efficiency of a simple gas turbine cycle is given in Section 18.9.1. All the first successful gas turbines made use of radial compressor technology: thanks to the centrifugal effect, this was able to produce a high-pressure rise more effectively than a multistage axial compressor at that time. The first functioning gas turbine was developed by Aegidius Elling (1861–1949). His first design from 1903 provided power in the form of compressed air from a radial compressor to drive other machines. The breakthrough in gas turbine technology leading to the modern jet engine for aircraft propulsion was made independently by Sir Frank Whittle (1907–1966) in England and Hans von Ohain (1911–1998) in Germany in the years just preceding the Second World War. Both of these inventors made use of radial turbocompressors in their first engines. The use of gas turbines for power generation also dates from this period, as the first marketable industrial gas turbine in the world was designed by Brown Boveri & Cie in 1939 for the Swiss city of Neuchatel, making use of axial compressors. The breakthrough for the gas turbine as an efficient power source for electricity generation only came during the last two decades of the twentieth century with the development of combined steam and gas turbine cycle power plant, operating on natural gas as a fuel. Such plants now achieve cycle efficiencies of over 60%.
More details with regard to the history of turbomachinery can be found in the books by Eckert and Schnell (Reference Eckert and Schnell1961), Jenny (Reference Jenny1993), Wilson and Korakianitis (Reference Wilcox1998), St. Peter (Reference Peter1999), Lüedtke (2004) and Eckardt (Reference Eckardt2014) and historical overviews of the development of centrifugal compressors are provided by Engeda (Reference Engeda1998) and Krain (Reference Krain2005).
1.5 Components of Radial Flow Turbocompressors
1.5.1 The Single-Stage Radial Flow Turbocompressor Stage
The compression process is carried out in a compressor stage which comprises four main elements: the inlet, the impeller, the diffuser and the outlet. These components are shown in Figure 1.7, which depicts a typical single-stage radial compressor, consisting of an intake with an inlet guide vane, an open (or unshrouded) impeller, a diffusing system (in this case a vaned diffuser) and a discharge volute. A single-stage machine with the front casing removed is shown in Figure 1.8.
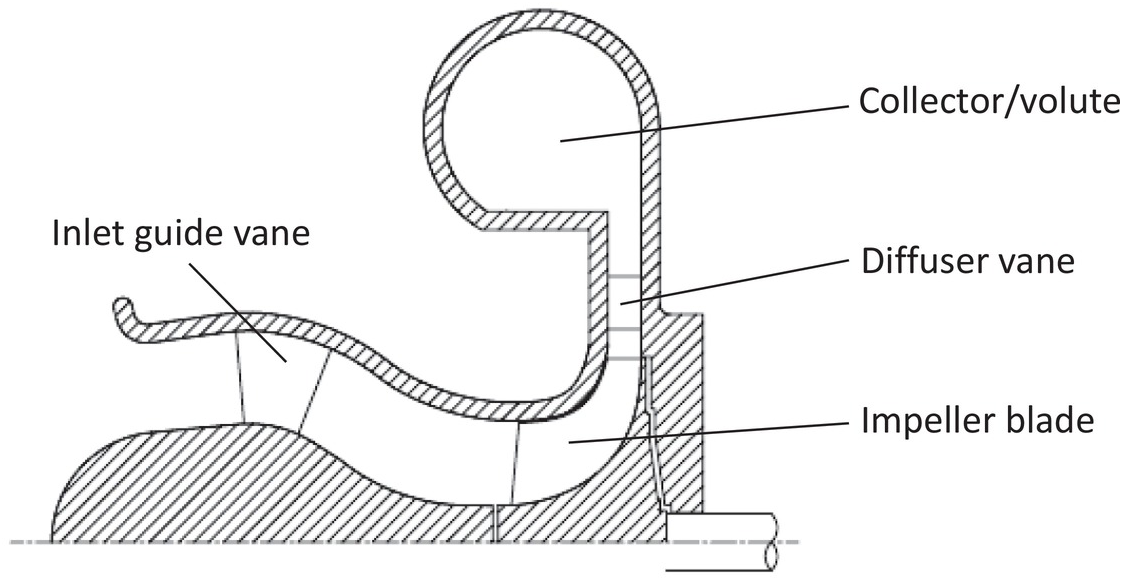
Figure 1.7 Sketch of a compressor stage components with a volute.
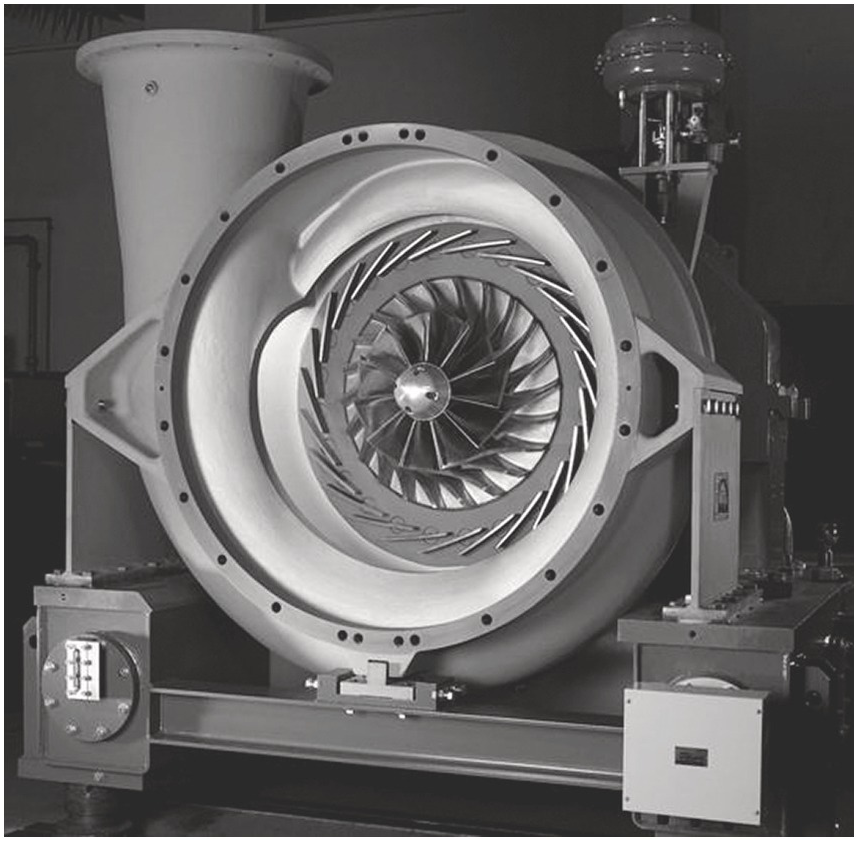
Figure 1.8 A single-stage compressor with a variable vaned diffuser and a volute with the inlet casing removed.
The inlet casing, inlet nozzle or suction pipe, accelerates the flow from the low velocities at the compressor flange, or from the surroundings, to the impeller inlet. It is important that this is done with the minimum of distortion to the velocity profile. There is no energy transfer; the total enthalpy remains constant, but losses occur. The inlet duct may be axial or radial and it may be fitted with variable inlet guide vanes to change the swirl component of velocity at the impeller inlet in order to provide a means of controlling the performance; see Section 17.5.4.
The impeller transfers energy to the fluid, raising both the pressure of the gas and its absolute velocity. In impellers with an axial inlet, the axial section of the blading at the front of the impeller is often known as the inducer. This axial element is rather like the inlet of an axial compressor and in the past was often treated as such. Industrial compressors often have the leading edge of the impeller in the axial to radial bend and do not have an inducer. The outlet of the impeller generally has backswept blades, which means they are leaning backwards away from the direction of rotation. Impeller design is considered in Chapter 11 of the book.
The diffuser converts the high kinetic energy at impeller outlet into a rise in static pressure. There is no further energy transfer from the shaft, so the stagnation enthalpy remains constant but the total pressure falls due to the losses. In some situations, with heat transfer from the surroundings, the total enthalpy may rise slightly, for example in a turbocharger compressor in close proximity to a turbine driven by the hot engine exhaust gases. The diffuser can be vaned or vaneless. In a vaned diffuser there is a small vaneless region upstream of the diffuser blades. Chapter 12 considers the design of the diffuser.
The outlet casing takes the fluid either to the outlet flange, to the inlet of a subsequent stage or to a combustion chamber. The scroll, volute or spiral casing collects the flow leaving the diffuser and brings it to the outlet flange. In many designs, a further reduction of kinetic energy is carried out downstream of the scroll by means of a conical outlet diffuser, as in Figure 1.8. In multistage (inline) compressor applications, the scroll is replaced by a crossover bend and a vaned return channel to remove the swirl velocity and direct the flow inwards into the inlet of the next stage, as shown in Figure 1.9. In gas turbine applications, there are often axial exit guide vanes to condition the flow for the combustor, which requires low swirl velocity, typically with a swirl angle less than 20°, and low absolute Mach number, 0.25 or less, as shown in Figure 13.10. Chapter 13 considers the design of the volute, the return channel and other stationary components of the gas path.
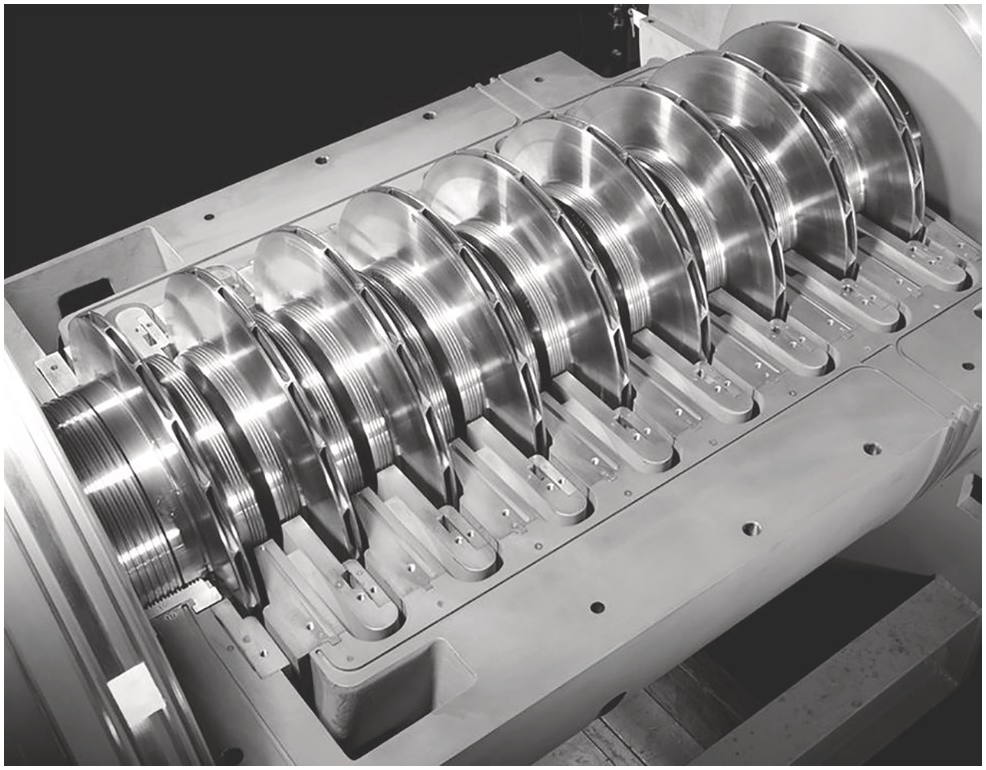
Figure 1.9 A nine-stage compressor with shrouded impellers.
1.5.2 Multistage Configurations
If a single-stage machine cannot reach a sufficiently high pressure, then multiple stages are used. Figure 1.4 shows the shaft of a three-stage compressor with open impellers. In this case, the lack of an impeller shroud cover requires that the mechanical design needs to include well-machined casing components to match with the impeller blade outer contour otherwise leakage flow over the tips of the blades lowers the efficiency. This leakage loss may be offset by the increased pressure ratio available due to the higher tip-speeds that are allowable when compared with shrouded impellers. Two-stage and three-stage compressors are possible with open impellers, but for more stages shrouded impellers are invariably used. The maximum number of impellers on a single shaft is approximately eight to ten. Figure 1.9 shows a process compressor with nine stages with a smaller outlet width of the impellers as the gas density increases.
1.5.3 Impeller Types
There are three broad categories that describe the different types of impeller. The first is whether the impeller is open or shrouded. The second is the value of the global flow coefficient, which is a nondimensional flow capacity defined in Chapter 8 and which determines the width of the flow channels needed. The third is whether the impeller has splitter blades between the main blades or not. Historically there is also a fourth distinction between impellers whose blades are radial at outlet and those with backswept blades. Nowadays nearly all impellers have some backsweep at the impeller outlet.
In single-stage applications, the impeller is usually unshrouded, or open, and has a high flow coefficient with an axial inlet, wide flow channels and three-dimensional blades with splitter blades, as shown in Figure 1.10, which shows an impeller with a low backsweep. There is usually no constraint on the axial length, so the impeller has an axial inlet with an inducer section. There is a small clearance gap between the tip of the rotating blades and the casing. As in all compressor applications, a relatively large clearance between the ends of the rotor blades and the casing has a detrimental effect on efficiency, and may also affect flow stability, such that the gap needs to be as small as practicable. In impellers with a diameter greater than 250 mm, the practical operating gap is typically 1/1000th of the impeller diameter but with a minimum size of between 0.5 and 1.0 mm.

Figure 1.10 The open impeller of a high flow coefficient stage with splitter vanes.
In multistage inline compressors, shrouded or closed impellers are most often used, as shown in Figure 1.11. This is because it is difficult to maintain such a small clearance between the impellers and the casing in all stages – the differential expansion between the rotor and the casing during startup causes an increase or decrease in the gaps and may lead to rubs between the impeller blades and the casing. In shrouded impellers axial labyrinth seals are used both in order to seal the flow over the shroud cover from the impeller outlet to inlet and also to resist leakage over the impeller backplate. In a multistage compressor, the volume flow decreases through the machine so that impellers of different flow coefficient are needed at different positions.
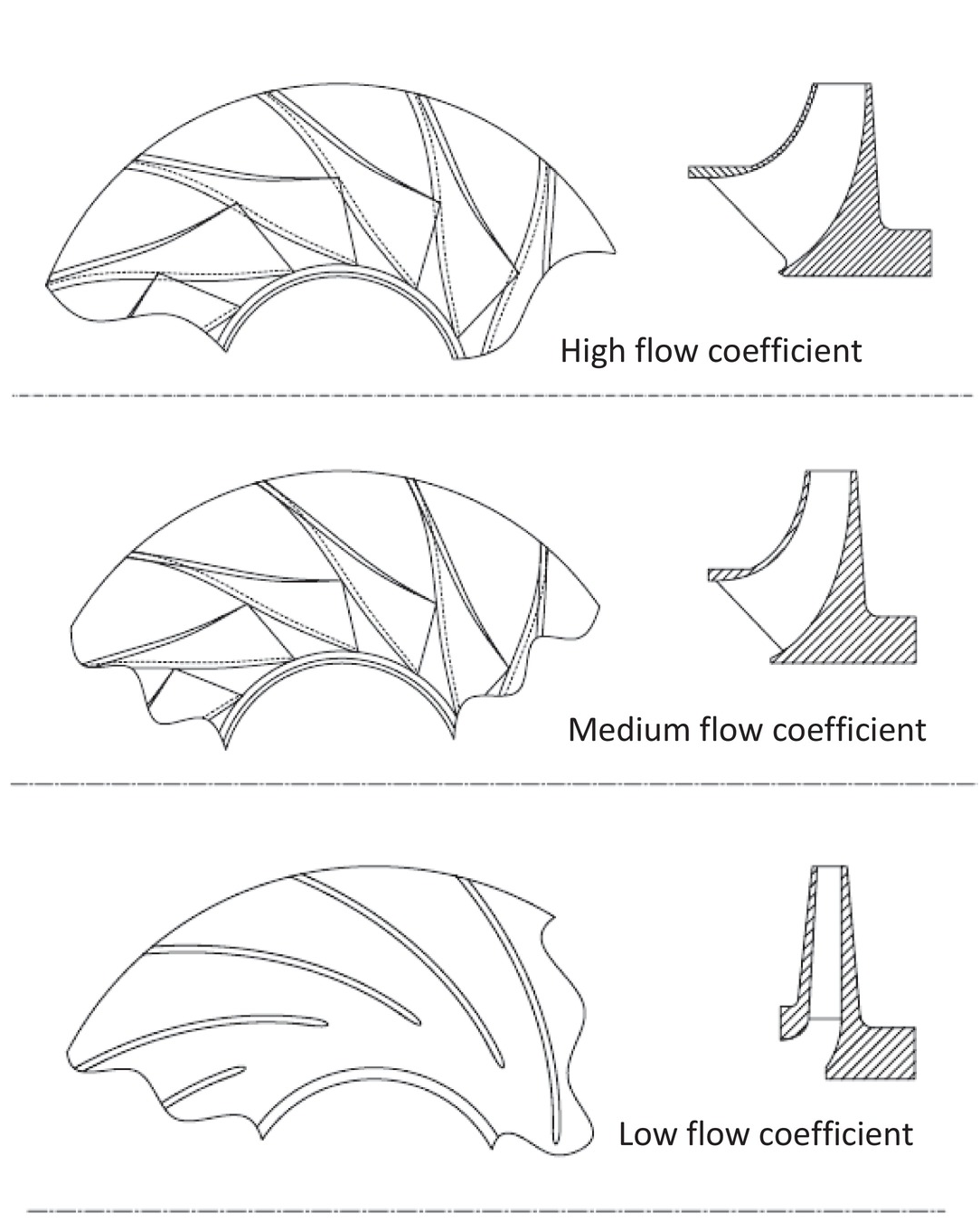
Figure 1.11 Shrouded impellers of high, medium and low flow coefficient process stages.
The leading edge of the impeller blades of multistage compressors is often inclined at an angle of about 30–45° to the radial direction in the axial-to-radial bend, in some cases even at 90°, that is, after the axial-to-radial bend. The reason for this inclination is purely mechanical. In multistage machines, thick shaft diameters are necessary for rotor stability. Since the root of the blade cannot be fixed to the shaft itself, the leading edge has to be inclined.
Shrouded impellers for high, medium and low flow coefficient process compressor stages are shown in Figure 1.11. High flow coefficient impellers are typically used in the inlet section of a multistage compressor and have three-dimensional blade shapes, an axial inlet and wide flow channels. Medium flow coefficient impellers have narrower flow channels, are shorter than those with high flow coefficients and are typically used downstream of the high flow impellers. They often have the leading edge in the bend to the radial direction and so do not have an axial inducer but still make use of three-dimensional blade shapes. Low flow coefficient impellers have narrow flow channels with the leading edge in a region of radial flow. They often have two-dimensional blades with a constant blade profile in the axial direction.
Shrouded impellers typically run at lower tip speeds than open wheels due to the higher mechanical stresses caused by the heavy shroud at a large radius, whereby the highest stress levels occur in the high flow coefficient impellers. However, shrouded impellers provide a more robust component that is less prone to blade vibration problems. As such, they are highly suitable for industrial and process applications.
In some industrial multistage compressors, the first stage may be positioned close to the axial thrust bearing, so that the impeller can be open but subsequent impellers are invariably shrouded. The absence of a cover means that open impellers can operate at higher tip speeds and thus produce greater pressure rise than shrouded stages. The disadvantage of open impellers forced to operate with a large clearance gap is their lower efficiency due to increased leakage flow between the blade tips and the casing. Furthermore, there is an increased risk of lower resonant blade natural frequencies resulting from the cantilevered attachment of the blades to the hub. Chapter 19 discusses mechanical design aspects (impeller stress, critical speeds and rotor stability) in more detail.
1.5.4 Features of Multistage Radial Turbocompressors
Multistage turbocompressors are mostly classified in relation to their mechanical design, method of construction and arrangement of the stages (Sandberg, Reference Sandberg2016). The main distinctions are between single-stage and multistage machines, which may be arranged in series or in parallel to meet the flow and head requirements of a specific application, as shown schematically in Figure 1.12. Single-stage designs are invariably overhung from the bearing with a single impeller placed outside of the bearing span, and in some cases two-stage configurations are also overhung designs. In some older applications with a very high-volume flow for the technology of the time, a double-suction configuration may be used with two impellers operating in parallel back-to-back on a single shaft with two inlets but only one outlet. In this configuration, both impellers are of the same design and may make use of a single diffuser, as shown in Figure 1.13.

Figure 1.12 Schematic diagram of different compressor configurations.

Figure 1.13 An early aero engine for propeller drive with a double-suction radial compressor, Rolls-Royce Nene developed in 1944.
The basic multistage configuration comprises several impellers aligned on a single shaft between two radial bearings, with the flow in the same direction within a single casing and with single inlet and discharge flanges. The limitation on the number of impellers allowed on a single shaft is determined by the rotordynamics of the long shaft, which in turn is strongly influenced by the axial length of the impellers and by the temperature rise, due to mechanical or process considerations; see Section 19.7.
The pressure profile around each impeller results in an unbalanced axial thrust force from the back disc towards the eye of the impeller. The sum of these forces for each impeller is counteracted by the combination of a balance piston at one end of the rotor and a thrust bearing. The balance piston is provided with a cross-sectional area where discharge pressure is imposed on one end of the piston and suction pressure on the other, partially negating the unbalanced axial force due to the impellers.
As an alternative to the straight-through inline configuration a back-to-back inline configuration may be used. The flow in the stages of the first section is then towards the centre of the machine, where the flows leaves the casing. It reenters at the other end of the shaft and then also flows towards the centre of the machine so that the inducers of the impellers in each section are oriented towards the shaft ends at both ends of the casing. This configuration can provide for external intercooling and mass flow addition or extraction between the sections. One advantage of the back-to-back design is its inherent characteristic to reduce, and roughly balance, the axial thrust force generated in the stages of each section. Since the two sections are oriented in the opposite direction to each other, unbalanced axial thrust forces from the individual sections act in opposite directions.
Another multistage compressor configuration is known as an integral gear compressor, shown in Figure 1.14. In its simplest form, a single impeller is directly connected to the end of a pinion which is driven by a bull gear. These impellers are often of an open design. More complex versions of the integrally geared compressor exist in which impellers are attached to both ends of the pinion (usually one of these impellers is open and one shrouded). Multiple pinions can be fixed to a single bull gear to develop a multistage machine. The rotational speed and size of each impeller can be optimised to enhance the overall efficiency of the compressor by using stages which are close to the optimum flow coefficient, as discussed in Chapter 10. In some process applications, one of the compressor stages on the pinion may be replaced by a turbine expander to recover process energy.

Figure 1.14 An integral gear compressor.
There are many applications where intercooling of the gas is required to maintain temperatures at acceptable levels or to provide the thermodynamic advantage of a compression process that is more nearly isothermal, as discussed in Section 4.7.4. Here it is possible to use multiple, single-section casings with intercooling provided between the casings. An alternative is to provide multiple sections within a single physical casing. In some applications, coolers are integrated into the casing of the compressor. These generally need to be specially adapted to particular applications, as shown in Figure 1.15.

Figure 1.15 An inline multistage compressor with two internal coolers.
Process compressors may require the addition or extraction of sidestream flows at some intermediate pressure of the overall application. The inlet and discharge flanges for the sideloads of each section must be attached to the casing. Adequate space is needed to accommodate these nozzles, which means that each section usually has at least two stages. For sidestream flows entering the compressor, special arrangements are needed in the casing to mix with the discharge flow of the preceding section. The sideload compressor is often used for refrigeration applications where the refrigerant gas can be obtained from economisers that operate at intermediate pressure levels in order to increase the overall efficiency of the refrigeration process.
Large pressure ratios cannot be handled by one casing alone as, for rotordynamic reasons, it is usually not possible to split the compression cycle into more than three sections within one casing. The major process compressor manufacturers therefore build compressor trains that may consist of up to four separate casings. The compressors are driven by a common driver, but gearing may be used between the stages to allow the sections to run at different speeds.
Additionally, there are two different modes of assembly for inline applications: horizontally and vertically split machines. In the horizontally split machines, which are used for low pressure service with up to about 75 bar, the casing is split horizontally along the centre line of the machine and the upper and lower halves are bolted together. Above this pressure, barrel compressors are used in which the horizontally split casing is inserted into a cylindrical casing which has a removable end cover bolted on the cylindrical casing. The increased strength available from the barrel casing allows these machines to be used up to 600–700 bar discharge pressure. Hanlon (Reference Hanlon2001), Brown (Reference Brown2005), Bloch (Reference Bloch2006) and Brun and Kurz (Reference Brun and Kurz2019) give many practical details. Some of the figures in the later parts of this chapter show some of the features discussed here.
1.6 Applications of Centrifugal Turbocompressors
1.6.1 Turbochargers and Superchargers
The largest application of radial compressors in terms of production numbers is in turbochargers, where around 25 million units are produced each year for automotive applications. Centrifugal compressors are used in turbochargers in conjunction with reciprocating internal combustion engines to provide an increased density of air for the engine. These are known as turbochargers if they are driven by the engine’s exhaust gas through a turbine; as turbosuperchargers if the compressor is mechanically driven by the engine or by an electric motor; and as turbocompound turbochargers if the turbine of the turbocharger is also mechanically linked to the engine shaft to recover power.
Turbochargers have been in use in marine diesel engines for nearly a century. Exhaust-gas turbocharging was the greatest single technical contribution to diesel engine progress helping the diesel engine to replace the steam turbine in marine propulsion. Turbocharging increases the power for a given engine size simply by forcing more oxygen into the cylinder so that more fuel can be burned. In the early days of the great oceangoing liners of the Norddeutscher Lloyd, Cunard and White Star Line, steam power was the preferred motive power. Kaiser Wilhelm der Grosse (1897) had two screws driven by quadruple expansion steam engines, the Lusitania (1909) had twin screws driven by Parsons steam turbines and the Titanic (1912) and her sister ships had triple screws, the outer two being driven by driven by triple expansion steam engines from Harland and Wolf exhausting into a Parsons low-pressure steam turbine for the central screw. The first application for large diesel motors in ships were the Preussen (1923) and Hansastadt Danzig (1926), both equipped with turbochargers, following their patented invention by Alfred Büchi in Switzerland in 1905. The high thermal efficiency of the turbocharged compression ignition engine gives high fuel economy, and the diesel engine became the primary power source for marine engines and for large engines for rail traction. Auguste Rateau extended the use of turbocharging to aircraft engines, allowing them to achieve higher power at altitude (Jenny, Reference Jenny1993).
Turbocharging of diesel engines is one of the most effective techniques for increasing their performance while reducing pollutants and fuel consumption (Galindo et al., Reference Galindo, Serrano, Margot, Tiseira, Schorn and Kindl2007). Turbocharging is essential for modern diesel engines and is becoming more so for gasoline engines. Turbocharging allows acceptable air-to-fuel ratios to be maintained with high injected fuel levels. The increase in the air density inside the cylinders enhances combustion as it is an efficient technique for accelerating the atomisation and mixing of the injected fuel and decreasing the penetration of the fuel jets inside the cylinders. During the 1980s, turbochargers became commonplace in medium-size diesel engines for trucks and lorries, and in the 1990s they began to be used for small automobile diesel engines. Here they were first deployed to provide more torque and power from a given engine and then, more recently, to allow motor downsizing – providing a smaller engine with improved power density and reduced emissions (see Figures 1.16 and 18.19). Latterly, turbochargers have become more commonplace on automotive gasoline engines for the same reasons.
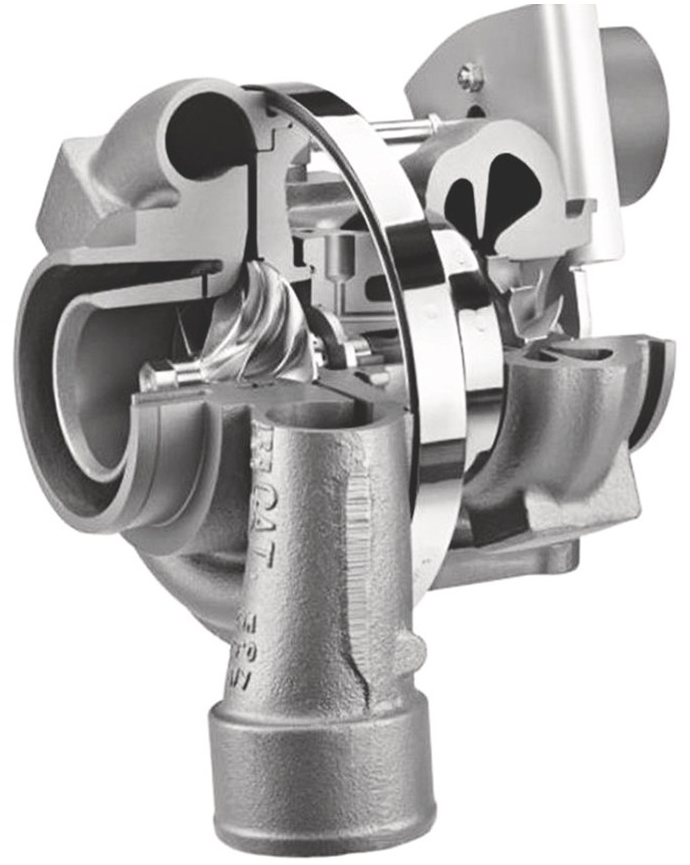
Figure 1.16 Radial compressor impeller in an automotive turbocharger driven by a radial turbine from the exhaust gas stream of the engine.
The key aerodynamic issues relating to the compressor in this application are the achievement of a wide operating range to match the variability of the engine flow at different speeds and power levels, and the maximisation of efficiency. Weak performance of a compressor in a turbocharged engine limits the behaviour of the drive train, particularly during transient conditions such as the drive-away process or acceleration from low engine speeds. These considerations come up against practical limits associated with compressor instability at low flow and the choking of transonic flows at high flow rates, both subjects covered in Section 18.10. In early automotive turbochargers, there was an issue of delayed transient response when power was needed which was related to the time taken for the turbocharger to accelerate to a suitable speed. This problem, known as turbo lag, has largely been solved using turbine bypass valves (wastegates), but higher efficiency is obtained with variable geometry turbines and, more recently, with multiple turbocharger arrangements with compressor stages in series or in parallel (Baines, Reference Baines2005) and improvements derived from reduction in inertia of the turbine.
Specific issues associated with small turbochargers are the high rotational speeds of above 250,000 rpm in small passenger car applications, combined with variable geometry turbines for the control of the matching of the turbine with the engine, as discussed in Section 18.10.6. At low engine speeds the stator (nozzle) vanes of the turbine are closed to reduce the flow capacity of the stage, which provides a high pressure drop at low flow producing sufficient power for the compressor to supply low-speed boost. At high engine speeds, the vanes are opened as otherwise there would be too much power and excessive boost for the engine.
Turbochargers have a large range of sizes; a turbocharger for a marine diesel engine may have an impeller diameter close to 1000 mm, and a turbocharger for a small gasoline engine may have a diameter of less than 40 mm. The compressor used in turbochargers needs to achieve a pressure ratio of between two and four in automotive applications and above six for larger diesel engines (for ships and power generation). In both cases, this pressure ratio is usually achieved by a single radial compressor impeller made of aluminium or titanium, but two-stage boosting systems are also sometimes used. Standards set by the industry for turbochargers have been established by SAE (1995). Ideal gas properties often work well to support the design, test and analysis of turbocharger centrifugal compressor performance.
1.6.2 Compressed Air
Compressed air at a pressure greater than atmospheric is required for many domestic and industrial purposes. It is used for power tools, such as air hammers, drills, wrenches and others and is also used to atomise paint and to operate air cylinders for automation. A study by Radgen and Blaustein (Reference Radgen and Blaustein2001) estimated that in Europe, 10% of all industrial electricity consumption is used in the production of compressed air. Typical system storage pressures are close to 1 bar (gauge), which means that a pressure ratio of two is required for the compressor, and a single-stage compressor as shown in Figure 1.8 is suitable.
1.6.3 Industrial Wastewater Treatment
Compressors are required in the treatment of sewage and wastewater. Air is needed to supply oxygen for the activation of bacteria to digest and break down waste material during the process. In addition, injecting air into a treatment tank provides a strong mixing and stirring movement of the fluid to keep solids and sludge in suspension in the liquid. A similar application is the provision of air for biochemical fermentation processes associated with yeast and pharmaceutical processes.
This is an interesting application as the air is forced into large digestion tanks with a fixed depth of water. The absolute pressure at the bottom of a 10 m tank is nearly 2 bar, and so a pressure ratio of two is needed to blow the ambient air into the water: a lower pressure rise is needed for shallower tanks. The same pressure rise is needed at different volume flow rates if the water depth remains the same. This is an interesting technical control problem for centrifugal compressors where the outlet pressure naturally increases at lower flows. The solution requires variable speed, variable guide vanes or variable diffuser vanes, or a combination of these features, as discussed in Section 17.5. Historically, machines used for this purpose were driven at constant speed through a gearbox by induction motors, but more recently advances in high-speed motor technology permit the use of direct drive with variable speed to assist in the achievement of broad range at constant pressure. Normally several compressors are available at any particular plant to give coarse increments in air supply. This drives the range requirement; each machine must be capable of operating at constant pressure to just below half of its maximum flow.
1.6.4 Air Conditioning, Air Extraction and Building Ventilation Applications
Ventilators are required to aerate and ventilate, to cool or heat processes and buildings and to dry products in many technical processes. The ventilators used range from large axial ventilators with a diameter of 20 m in forced convection cooling towers to small axial ventilators with a diameter of just a few centimetres as in a conventional laptop. Centrifugal machines are used where higher pressures are needed and, in these applications, they are sometimes called blowers.
There are several special forms of design in this market. The designs are generally at low absolute pressure levels and low pressure rise but with low blade speeds – mainly to avoid noise, which increases with the sixth power of the speed. This results in relatively high nondimensional aerodynamic loading (see Section 7.5), hence the attraction of a centrifugal stage. These machines superficially appear to be less technically advanced than other high-pressure radial compressor applications: as in many low-speed applications, there is no diffuser, but the impeller discharges directly into the volute casing. Nevertheless, the requirement of low speeds to avoid noise, and the general task of keeping costs as low as possible, leads to substantial design challenges.
1.6.5 Vacuum Compressors
In addition to providing compressed air, compressors can be used to evacuate air and so reduce the pressure in an enclosure. Examples can be found in machines developed for dewatering of pulp felt on paper, board and tissue machines. The example shown in Figure 1.17 has several different inlet streams along. the axis to match the suction and vacuum requirements at different positions of the paper machine. Air at the highest vacuum level is compressed in the first stage of the blower and ducted via return channels to the second stage, where it is mixed with air from the medium vacuum level in the first sidestream. This process is repeated in the third and fourth compression stages. The design is such that four different vacuum levels can be generated in one unit. Thanks to the additional inlet flow upstream of each stage, the flow coefficients and the outlet width of successive stages do not reduce towards the end of the compressor but at first increase through the machine.

Figure 1.17 A vacuum blower with multiple sidestreams for dewatering paper pulp.
Similar applications with low inlet pressure requirements are found in vacuum cleaners, albeit on a much smaller scale using small single-stage compressors at high speed; see Section 1.6.19.
1.6.6 Vapour Compression Refrigeration and Heat Pumps
Refrigeration plants transfer heat to or from processes and buildings. The vapour cycle refrigeration process is the most common method of doing this. The vapour compression thermodynamic cycle is similar to a heat engine cycle but works in reverse. In the basic cycle, the liquid refrigerant at low pressure is evaporated to cool the evaporator at low temperature, then the evaporated gas is compressed to a higher pressure to the condenser, where heat is removed by cooling the gas to the liquid state. The liquid is then throttled back to the evaporator pressure through a valve. This is the standard process in a domestic refrigerator where the evaporator removes heat from within the enclosed space. Where the flow rate is small, reciprocating compressors are used. In larger heat pumps, it is the heat transferred to the condenser which is of interest for heating purposes. The evaporator can make use of different sources of heat for the evaporation (ambient air, rivers, lakes or the ground). The electric-driven heat pump is one of the most promising technologies in term of reducing greenhouse gas emissions in the future as it is an energy efficient way to provide space heating provided the power is from renewable sources (Schiffmann, Reference Schiffmann2008).
On a larger scale, a wide variety of vapour compression cycles are used to improve the coefficient of performance, involving multistage compressors with liquid suction heat exchangers, flash gas economisers, heat exchange economisers and cascade refrigeration systems with multiple refrigerants (van Gerner, Reference Van Gerner2014). The major focus today is the effective use of synthetic refrigerant gases that are not damaging to the environment. There is a wide range of new working gases (refrigerants) becoming available for application at different temperature levels. Carbon dioxide (CO2) has been widely investigated for use as a working fluid in refrigeration cycles: it is inexpensive, nonexplosive and nonflammable and has both no ozone-depleting potential (ODP) and low global warming potential (GWP) compared to other refrigerants.
Centrifugal compressors are used in a wide range of sizes and configurations for larger heat pumps and refrigeration applications. The use of refrigerants means that real gas properties are needed to design, test and analyse the performance of these machines. The gases generally have a high molecular weight and consequently a low speed of sound, such that large pressure ratios and transonic flow occur even though the blade speeds remain moderate compared to compressors operating with air. Standards set by the industry for these compressors include those published by the American Society of Heating, Refrigerating and Air-Conditioning Engineers (ASHRAE), the American Society of Mechanical Engineers (ASME), the American Petroleum Institute (API) and the European standard EN-14511.
Air cycle refrigeration is a form of gas refrigeration in which air is used as the working fluid and does not change its state but remains as a gas throughout the process in a reversed Brayton or Joule cycle. If air is used as the working fluid, a closed system is not required and the cooled air can be used directly. This technology is used in most modern aircraft cooling applications for the supply of cabin air, in wing ice protection systems and in larger transport systems, such as train air conditioning and for cooling of electronics. An air cycle machine typically includes a centrifugal compressor and one or two radial expansion turbines. The air from the compressor is first cooled by a heat exchanger, then expanded through a turbine, thus producing cold air. In an aircraft cooling and ventilation system, there are often two radial turbines, a radial compressor and an axial fan all on the same shaft.
1.6.7 Gas Turbines for Power Generation
In power generation, the main application of gas turbines is in combined cycle gas and steam turbines, where the hot exhaust gases from the gas turbine (at around 600°C) are used in a heat recovery steam generator to produce steam for a so-called bottoming cycle steam power plant. Such high-power compressor applications need a large flow capacity and are invariably equipped with axial compressors. Centrifugal compressors, both in single and two-stage configurations, are suitable for application to relatively small simple-cycle gas turbines in the 2–5 MW power range at pressure ratios typically between seven and eleven.
The first gas turbine to provide a net power output was that of Aegidius Elling which made use of a six-stage centrifugal compressor with water injection as a means of intercooling (Bakken et al., Reference Bakken, Jordal, Syverud and Veer2004). The impeller was a radial bladed design and looks quite dated compared to modern designs, but the stage used vaned diffusers and the shape of these look quite modern in form, as can be seen by comparison of those in Chapter 12 (see Figure 1.18). Many other images of this machine are available on the website of the Norway Museum of Science and Technology.

Figure 1.18 An i.mpeller and diffuser from a radial compressor stage of the 1904 gas turbine of Aegidius Elling.
In more recent years, small micro gas turbines (MGT) for distributed power have been developed, based largely on turbocharger technologies making use of natural gas to provide local sources of power and heat (Moore, Reference Moore2002). These smaller machines make use of radial flow turbocompressors in a regenerative gas turbine cycle and are becoming widespread in distributed power and combined heat and power applications. They range from handheld units producing less than a kilowatt, to commercial-sized systems that produce tens or hundreds of kilowatts. Microturbine systems have many advantages over reciprocating engine generators, including a higher power-to-weight ratio, lower emissions and the fact that they have only one main moving part.
Normal gas turbine cycles are open and make use of air as the working fluid such that the combustion products pass directly through the turbine. An active area of research is the development of closed cycle Brayton power units employing supercritical CO2 with high density as the working fluid and radial compressors to pressurise the gas (Conboy et al., Reference Conboy, Wright and Pasch2012). Supercritical CO2 is a fluid state of carbon dioxide held above its critical point (i.e., critical pressure and temperature). The density is similar to that of a liquid and allows for the pumping power needed in a compressor for a given pressure ratio to be significantly reduced, as discussed in Section 2.8.2. Such cycles could provide significant efficiency gains in geothermal, coal, nuclear and solar thermal power production.
1.6.8 Automotive Gas Turbines
One almost obsolete application, included for completeness, is automotive gas turbines, which, because of their relatively small size and power requirement, made use of radial turbocompressors in a regenerative gas turbine cycle. Prototypes were developed by Allison, Chrysler, Daimler, Rover, Austin and others in the middle of the last century. The poor throttling response from the high rotational moment of inertia and the low thermal efficiency in such small machines given their low pressure ratio, plagued the further development for this application (Walsh and Fletcher, Reference Walsh and Fletcher2004).
The automotive relevance of radial compressors in small gas turbines has recently been revised: small micro gas turbines can be used to provide power to charge the batteries of hybrid or electric vehicles and so act as range extenders. As the gas turbine is not directly connected to the vehicle wheels, it can operate intermittently at its natural high rotational speed without throttling.
1.6.9 Jet Propulsion Gas Turbines
Over 25,000 gas turbines are in operation as aircraft jet engines, many of them in military applications. The key development in this area is towards lighter and quieter machines with lower specific fuel consumption and lower emissions. Normally a key criterion for engines for aviation propulsion is high flow per unit frontal area, and this tends to favour the axial compressor for most civil transport and modern military applications. In addition, at the physical size typically needed for aero engines in large passenger aeroplanes, the multistage axial compressor normally has an efficiency advantage over a centrifugal at a similar overall pressure ratio.
Historically, however, radial compressor technology was used in all early jet engines, for example in both of the first two turbojets, the Whittle engine of 1937 (Whittle, Reference Whittle1945) and the Ohain engine of a similar date (Von Ohain, Reference Von Ohain and Peter1989). This was partly due to the advantage given by the centrifugal effect producing compression via radius change. Furthermore, experience with suitable centrifugal stages had already been accrued at that time in the supercharging of piston engines for aircraft propulsion, such as the Rolls-Royce Merlin engine of the Spitfire aircraft. Figure 1.13 shows an early engine of this period. The advantage of the centrifugal compressor stage was the relative ease with which an adequate level of pressure ratio and efficiency could be reached. The requirement for high throughflow in the gas turbine was initially achieved by applying a double suction impeller, with two inlets and a single outlet. A double suction impeller, as shown in Figure 1.13, has no back-plate and so in this configuration there is the added advantage that there are no parasitic disc friction losses; see Section 10.6.2.
Major contributions to aerodynamic theory were made in the 1940s and 1950s, and these led to the development of satisfactory axial compressors with more power and higher efficiency, and axial compressors gradually became the standard designs for larger aeroengines (Nichelson, Reference Nichelson1988). However, in the earliest versions of the first passenger jet aircraft, the de Havilland Comet, four Rolls-Royce Ghost engines with a single-stage centrifugal impeller were used. The Comet engines were later replaced by the Rolls-Royce Avon engine with a multistage axial compressor. Since that time, centrifugal compressor technology has only been retained for smaller aeroengines found, for example, in business jets, helicopters, drones and in auxiliary power units (APU). The specific applications with different engine architectures and power requirements necessitate different types of centrifugal compressor design (Rodgers, Reference Rodgers1991; Lou and Key, Reference Lou and Key2019). Cross-sectional images of many different gas turbine configurations using centrifugal compressors are given by Grieb (Reference Grieb2009).
One important application of centrifugal technology is in turboshaft engines for helicopters and propeller-driven aeroplanes. These may use a low-flow coefficient centrifugal stage for the final compression stage downstream of a multistage axial compressor on the same shaft, shown in Figure 1.19, known as an axicentrifugal configuration. The radial compressor in an axicentrifugal compressor has a very high hub radius compared with other radial machines designed for higher flow coefficients. At intermediate and lower thrust levels, a turboshaft engine may incorporate a single-stage centrifugal compressor, shown in Figure 1.19, or a two-stage centrifugal compressor with open impellers, as shown in Figure 1.20.

Figure 1.19 The rotor of an axicentrifugal compressor with a low-flow coefficient impeller and the rotor of a high-flow coefficient impeller for helicopter engines with a single radial compressor stage of a similar duty.

Figure 1.20 A cutaway view of the shaft of the MTR390 gas turbine engine with two centrifugal compressors.
In the ongoing quest for reduced specific fuel consumption (SFC) and emissions, the thermodynamic cycles of gas turbines for aircraft application are pushed towards increased overall pressure ratio and a higher bypass ratio. The ramification for the core engine is a reduction in the volume flow at compressor delivery. For axial stages, this implies a high hub tip ratio, reduced blade heights and stronger effects of clearance which erode the efficiency benefit over centrifugal stages. Recent trends have seen axicentrifugal machines considered for engines for smaller regional jet transport aircraft, where at this smaller size a high efficiency centrifugal stage can replace four or five lower efficiency axial stages.
An example is given in Figure 1.21. This involves a high-flow coefficient centrifugal stage as the high-pressure compressor on the high-speed spool of a twin-spool jet engine, following the fan and a low-pressure axial compressor (also called a booster) on the low-speed spool. In this case, there is a swan-neck duct downstream of the booster to bring the flow down to the radius of the impeller eye so the impeller is not constrained to have a thick shaft. Larger engines remain all axial. The architecture for more adventurous cycles, which divert core flow through heat exchangers in the bypass duct to benefit from an intercooled compression process, as discussed in Section 4.7.4, may also suit a final centrifugal stage in the core engine.
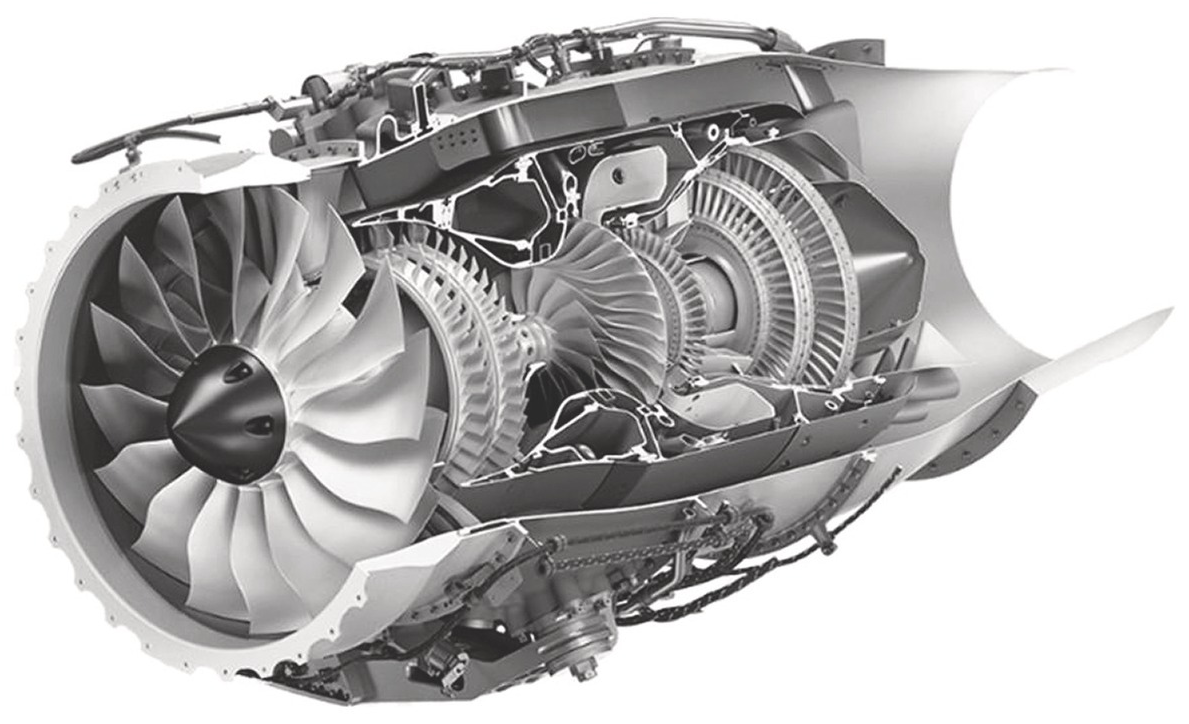
Figure 1.21 A cutaway view of the HF120 twin-spool aero engine for a business jet with a centrifugal compressor on the high-speed spool.
1.6.10 The Auxiliary Power Unit
The APU is a small constant-speed gas turbine engine located in an aircraft’s tail used to run an electric generator and provide pressurised air during operation on the ground. The design of such devices forces the choice of high-flow coefficient impellers to minimise the size. A cutaway view of the shaft of an APU of the type used in many Airbus aeroplanes is shown in Figure 1.22. The impeller on the left is part of the APU gas turbine, and the impeller on the right is known as the load compressor and provides compressed air output at a pressure ratio of four. The compressed air feeds the aircraft air conditioning system via pipes running through the fuselage and may also be employed in an air turbine starter to spin the main engines during starting.
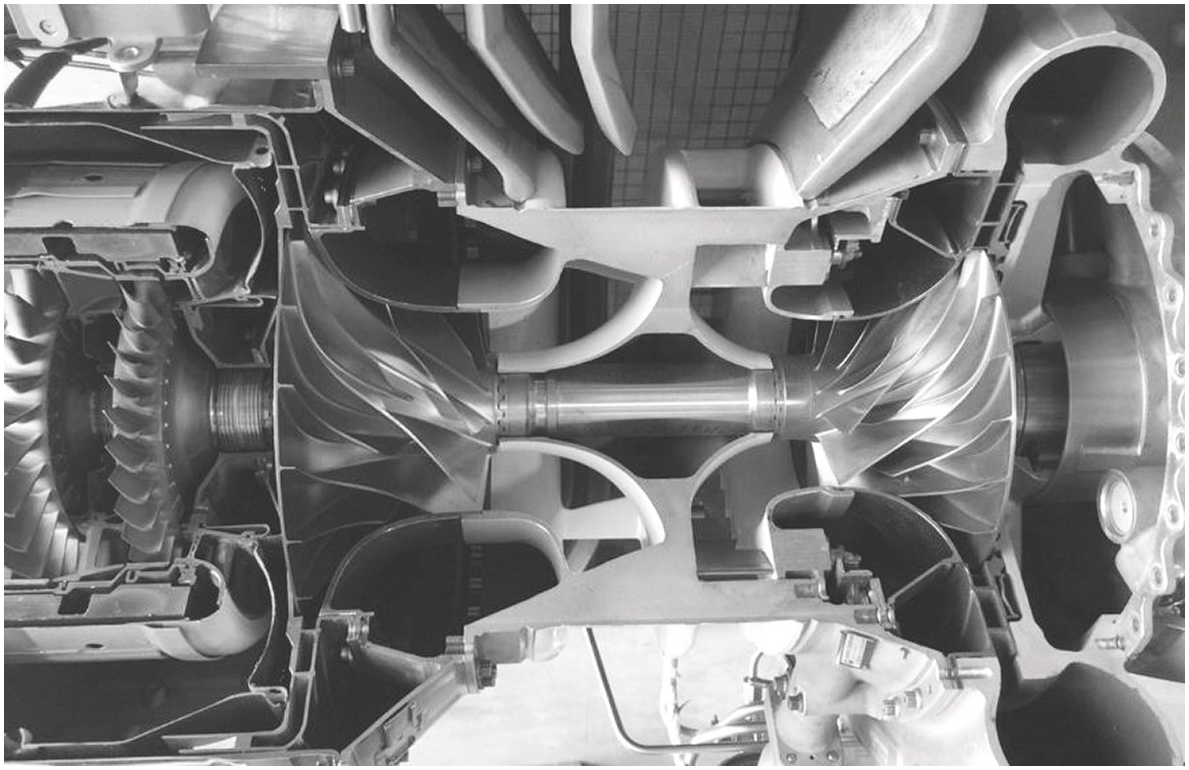
Figure 1.22 A cutaway view of the shaft of an auxiliary power unit with two centrifugal compressors driven by an axial turbine.
1.6.11 Oil, Gas and Chemical Applications
There are innumerable applications of radial compressors in the oil, gas and chemical industries, and an excellent description is given by Brun and Kurz (Reference Brun and Kurz2019) and a summary given here.
Pipeline compressors are installed along natural gas pipelines in compressor stations at intervals of roughly 100 km to pressurise the gas and so aid its transport along the pipeline. Typically, they make use of single-stage or multistage centrifugal compressors driven by a natural gas fired gas turbine, making use of the fuel in the pipeline. The special requirements in this application are high flow stages with high efficiency and a wide operating range.
Centrifugal compressors for natural gas processing, petrochemical and chemical plants are often single-shaft multistage machines driven by large steam or gas turbines. Their casings are often horizontally split at pressure levels below 100 bar, as shown in Figure 1.23, or are of the barrel type at high pressure levels, where a horizontally split unit is inserted into a vertically split casing acting as a pressure vessel, as shown in Figure 1.24.

Figure 1.23 A horizontally split compressor with with two inline sections and a sidestream entering after two stages.

Figure 1.24 A barrel compressor showing the horizontally split internals extracted from the barrel casing.
Standards set by the industry (ANSI, API, ASME) result in large, thick casings to maximise safety. The impellers are mostly, if not always, of the covered style with a shroud attached to the blades. This type of compressor is also often called an API-style compressor, from the American Petroleum Institute standard API 617 (2002). Use of real gas properties is needed to properly design, test and analyse the performance of natural gas pipeline centrifugal compressors, and great care must be taken in the matching of the stages.
There is increasing industrial activity in the compression and liquefaction of natural gas (CNG and LNG) and ethylene. These more unusual working fluids have high molecular weights and low sonic velocities leading to high Mach numbers. This causes the performance maps to be very narrow with a limited range between surge and choke, exacerbating the difficulty in matching stages. Another design issue is that in many applications these compressors have sidestreams to accommodate flows entering or leaving the compressor at intermediate pressures. These pressures depend on the process for which they were intended or the requirements of intercooling. The standard approaches that have been used for machines working with air are no longer applicable to these compressors, and the importance of the Mach number on performance is given strong emphasis in the analysis presented by the current authors.
A range of different compressors are used in these processes as follows:
The feed gas compressor compresses natural gas received at the plant to the required pressure for the condensate removal process.
The flash gas compressor repressurises the vapour produced during the condensate removal process.
The off-gas compressor compresses natural gas to the required pressure needed for acid gas removal, mercury removal and dehydration.
The regeneration gas compressor feeds the main cryogenic exchanger with required refrigerant.
The CO2 injection compressor is used to inject carbon dioxide into a storage well.
The lean gas compressor raises the lean natural gas pressure to a level suitable for the main cryogenic exchanger.
The end flash gas compressor pressurises the vapour produced during the refrigeration process.
The boil-off gas compressor repressurises boil-off gas for re-liquefaction or use in this gaseous state.
The N2 booster compressor adjusts the calorific value of the gas being sold.
The fuel gas compressor or fuel-boost compressor elevates the pressure of the gas stream to the inlet pressure required by the turbine combustion chamber.
Gas reinjection compressors are used for the reinjection of natural gas into an underground reservoir to increase the pressure in the chamber, decrease the viscosity of the crude oil and increase the flow of oil to production, leading to enhanced oil recovery (EOR). Centrifugal compressors for such uses are often single-shaft, multistage machines driven by gas turbines. With discharge pressures approaching 700 bars, casings are of the barrel style, and the stages are special designs of low flow capacity with narrow flow channels.
In some applications, the gas recovered from the oil well contains significant amounts of hydrogen sulphide. The gas can be reinjected directly into the well without removing the hydrogen sulphide (so-called sour gas) and without removing the acid content such as carbon dioxide (acid gas). In oil refineries or natural gas processing plants, the removal of hydrogen sulphide and other compounds is referred to as ‘sweetening’ as it removes the sour smell of the gas. Similar applications involve the injection of CO2 into oil wells. This is particularly used for compressors in subsea gas recovery applications, which are usually hermetically sealed and oil-free with magnetic bearings and direct-drive integrated motors (Fulton et al., Reference Fulton, Klein, Marriott and Graham2001).
1.6.12 Fluid Catalytic Cracking Compressors
The growing demand for light hydrocarbons is driven by the worldwide need for kerosene, diesel oil and gasoline as combustion fuel in many transport industries and the need for benzine or propylene for plastic or synthetic fibres. The fluid catalytic cracking (FCC) process refines various heavy hydrocarbons to lighter, more valuable products via high-temperature catalytic cracking. In the first part of the process, high molecular weight hydrocarbons are cracked in the presence of a catalyser with air at high temperature and pressure, often supplied from a large axial-centrifugal compressor.
1.6.13 Air Separation Plants
Air separation plants separate air into its constituent components, such as nitrogen, oxygen, argon, helium and other inert gases. The process is known as cryogenic distillation, and the air must first be cooled and liquefied using the Joule–Thomson effect. Air is first compressed to typically between 5 and 10 bar in a multistage turbocompressor and cooled before starting the expansion process. The compression work is minimised by using intercooling to try to approximate an isothermal (constant temperature) process, as discussed in Section 2.7.4. The cooling can be internal to the inline compressor (see Figure 1.13), or the compressor may have external coolers and comprise multiple single stage compressors driven by a bull gear known as integral gear compressors (see Figure 1.12). The cooler can also be in the form of cooling jackets embracing the compressor flow channel. A brief history of intercooled compressors with internal cooling, going back to a machine with a water-cooled casing in 1906, is given by Strub (Reference Strub1974).
1.6.14 Synthetic Fuels, Coal Liquefaction and Gas to Liquid
The Fischer–Tropsch process describes a series of chemical reactions that convert a mixture of carbon monoxide and hydrogen into liquid hydrocarbons, which are then used as synthetic lubrication oils and synthetic fuels. These reactions occur in the presence of appropriate catalysts, typically at temperatures between 150 and 300°C and pressures of up to several tens of atmospheres, which means that suitable compressors are needed. The process was first developed in Germany (Stranges, Reference Stranges2000), and was used during the Second World War to provide liquid fuels from coal. It now serves as an important reaction in both coal liquefaction and gas-to-liquids technology as well as many other chemical processes aimed at producing compounds based on hydrocarbon chains from coal. In the decarbonised energy system of the future, synthetic fuels from renewable electricity are likely be an important supplement to the direct use of electricity from renewables.
1.6.15 Carbon Capture and Storage (CSS)
This gas reinjection technology may in the future be applied to reinjection compressors for subterranean sequestration of CO2 in carbon capture and storage systems. The technology reduces greenhouse gas emissions from thermal power plants by injecting CO2 into depleted oil and gas fields or in to other suitable geological formations. The pressure level required for reinjection is above 200 bar. Very large machines will be needed to deal with large volumes of CO2.
1.6.16 Compressed Air Energy Storage
The exploitation of wind energy is an efficient way to reduce dependence on fossil fuels and reduce global warming, However, wind power is intermittent in nature. Compressed air energy storage (CAES) is a technology which uses available electrical power in times of surplus power to compress air and store it in a large void above or below ground. The stored air is then reheated and expanded through expanders which are attached to generators which convert the stored energy to electricity (Berman, Reference Berman1978). In large systems, axial turbomachinery may be used, but in smaller systems radial turbomachinery technology may be appropriate (Zhang et al., Reference Han, Gua and Yand2017).
1.6.17 Centrifugal Steam Compressors for Mechanical Vapour Recompression
Thermal separation processes, such as evaporation and distillation, are energy intensive and often make use of mechanical vapour recompression systems. In a conventional evaporator, the vapour stream produced is condensed, meaning that most of its energy content is lost. A mechanical vapour recompression (MVR) system compresses the vapour to a higher pressure and therefore acts like a heat pump, adding energy to the stream which can be recovered at a higher temperature. The elevated temperature enables the use of the compressed vapour to heat the evaporator. In mechanical vapour recompression, a turbofan or blower (either single-stage or multistage) is used to compress the whole vapour flow to a pressure that is sufficient to heat the evaporator. In this way, the evaporator does not consume steam during operation.
The main fields of application are currently in the food and beverage industries (evaporation of milk, whey and sugar solutions), the chemical industry (evaporation of aqueous solutions), salt works and desalination (evaporation of saline solutions) and environmental technology (concentration of wastewater). Single-stage centrifugal compressors with impellers made of standard materials, for example steel, are capable of achieving a water vapour pressure increase of a factor of 1.8. If higher-quality materials such as titanium or multistage compressors are used, the vapour pressure increase may be up to 2.5. The technical challenges for this application are the high tip-speeds needed because of the high speed of sound in steam – which is around 475 m/s – and the erosion by water droplets, if the vapour falls below the saturation line.
1.6.18 Fuel Cells
Research and development of fuel cell systems for many applications has dramatically increased in the past few years. In particular, the use of fuel cells running on hydrogen in vehicles with an efficiency close to 50% could replace internal combustion engine running on gasoline or diesel fuels with an efficiency of 20–25%. This leads to a number of unique challenges, such as safety, physical packaging within the vehicle, durability and operation under extreme environmental conditions. In addition, there are demanding duty cycles that include high peak power requirements and a rapid response time (Zheng et al., 2008). Increasing the pressure of the fuel cell system can give higher efficiency, higher power density and better water balance characteristics for the fuel cell. The centrifugal compressor, driven by an auxiliary power source or from a turbine in the fuel cell exhaust, offers higher power with good system efficiency with the advantages of small volume, low cost and high reliability for this innovative application (Berenyi, Reference Berenyi2006).
1.6.19 Microcompressors
New applications of small centrifugal compressors are associated with the development of high-speed motors allowing the rotor size for a similar mass flow and pressure ratio to be reduced. The application of ultra-high-speed miniature centrifugal compressors with an impeller diameter less than 30 mm and the use of ultra-high-speed electric motors to provide rotational speeds between 200,000 and 600,000 rpm are now possible. The enabling technology is the use of high-speed permanent-magnet synchronous motors up to 1,000,000 rpm, whereby the rotor is a diametrically magnetised samarium cobalt (Sm2Co17) permanent magnet which is encased in a retaining titanium sleeve.
Electromagnetic, thermal, rotordynamic and mechanical design are all a challenge for such machines. In a growing number of applications at low flow rates, such microcompressors can be successfully used to replace much larger positive displacement devices (such as liquid ring compressors) or to replace larger centrifugal compressors operating at lower rotational speeds (Casey et al., Reference Casey and Robinson2013). A common application is in high-speed compact vacuum cleaners. A range of small impellers with different flow coefficients and diameters for such compressors is shown in Figure 1.25. There are also concepts for microgasturbines capable of achieving a high power density compared to batteries using compressor impellers with an impeller of 8 mm rotating at 1.2 million rpm at design (Epstein, Reference Epstein2004; Sirakov et al., Reference Sirakov, Gong, Epstein and Tan2004).

Figure 1.25 Ultra-high-speed radial microcompressor impellers of different sizes and flow coefficients.
1.6.20 Medical Applications
At the lowest level of pressure rise and flow, centrifugal compressors and ventilators are becoming increasingly common in medical applications. They are used as the source of air supply for small continuously positive airway pressure (CPAP) devices for the treatment of sleep apnoea and in the provision of ventilation air for patients with critical lung infections. These particular devices must operate as quietly as possible. This means that although the power requirement for the flow and pressure rise necessary for even a large patient is modest, high efficiency and relatively high loading and low tip-speed to support low broadband noise are needed.
1.7 Some Other Publications
1.7.1 Books
There are many useful publications offering complementary cover of similar or related technical areas as this book. For completeness, a very short description of the most relevant books on radial compressors is given here.
Aungier, R. H. (Reference Aungier2000) Centrifugal Compressors – a Strategy for Aerodynamic Design And Analysis. ASME Press
A useful guide to practical aspects and to design guidelines for radial flow industrial compressors but less depth on the theoretical aspects and high-speed applications.
Bloch, H. P. (Reference Bloch2006) A Practical Guide to Compressor Technology. Wiley
Not theoretical, mainly intended for users and operators close to real applications.
Boyce, M. (2003) Centrifugal Compressors: A Basic Guide. PennWell
Not theoretical, mainly intended for users and operators close to real applications.
Brown, R. N. (Reference Brown2005) Compressors: Selection and Sizing. Elsevier
Not theoretical, mainly intended for compressor users. Includes all types of compressors.
Brun, K. and Kurz, R. (Reference Brun and Kurz2019) Compression Machinery for Oil and Gas. Gulf Professional Publishing.
A useful and practical up-to-date review of centrifugal, reciprocating and screw compressors in oil and gas applications, with multiple contributors from industry and academic research.
Cumpsty, N. A. (Reference Cumpsty2004) Compressor Aerodynamics. Krieger Publishing Company
A useful theoretical introduction to both axial and radial compressors but concentrating mainly on axial machines. Very strong on research issues, less pragmatic advice offered on design aspects.
Eckert, B. and Schnell, E. (Reference Eckert and Schnell1961), Axial- und Radial-Kompressoren, Springer, Berlin.
A good section on radial compressors but in German, out of date and out of print.
Ferguson, T. B. (Reference Ferguson1963) The Centrifugal Compressor Stage, Butterworth, London
A logical, well-written introductory book on basic principles, but out of date and out of print.
Gresh, T. M. (Reference Gresh2018) Compressor Performance. Butterworth Heinemann, Elsevier.
A useful book explaining the practical aspects of aerodynamics of compressors for equipment users involved in selecting, monitoring and maintaining various types of compressors; uses American units.
Gülich, J. F. (Reference Guo, Zhang, Xu, Zheng and Zhuge2008) Centrifugal Pumps. Springer, Berlin.
A good and wide-ranging book on centrifugal pumps with good design guidelines, some of which are useful for radial compressors.
Hanlon, P. C. (Reference Hanlon2001) Compressor Handbook. McGraw-Hill.
Not at all theoretical, mainly practical and intended for compressor users. Includes all types of compressors and describes many applications from many different contributors.
Japikse, D. (Reference Japikse1990) Centrifugal Compressor Design and Performance. Concepts/NREC.
A compendium of knowledge giving an introduction to centrifugal compressors and including useful information on the design of the various components.
Lüdtke, K. H. (2004) Process centrifugal compressors. Springer.
An excellent book on industrial multistage process compressors with useful design guidelines and practical information on these machines.
Traupel, W. (Reference Traupel1962) Die Theorie der Strömung durch Radialmaschinen. G Braun.
An interesting description of the physics of radial compressors as understood in the early 1960s; in German and out of print.
Van den Braembussche, R. (Reference He and Zheng2019) Design and Analysis of Centrifugal Compressors. ASME Press, Wiley.
A useful up-to-date book with some excellent complementary information, especially on secondary flows, vaneless diffuser instability, asymmetry from the volute flow and optimisation methods.
Watson, N. and Janota, M.S. (Reference Watson and Janota1982) Turbocharging the Internal Combustion Engine. Macmillan.
Despite being out of date, this includes an excellent introduction to radial flow compressors in the context of turbocharging; a reprint has recently been issued.
Whitfield, A. and Baines, N. C. (Reference Whitfield and Baines1990) Design of Radial Turbomachines. Longman, John Wiley and Sons.
A useful and pragmatic description of the technology and design methods for both centrifugal compressors and radial turbines working in compressible flow.
1.7.2 Technical Conferences and Journals
The major sources of up-to-date technical literature for radial compressors are the following technical conferences:
The ASME/IGTI Turbo Expo. The Turbomachinery Technical Conference and Exposition, organised annually by the ASME International Gas Turbine Institute (IGTI). The conference hosts an enormous number of turbomachinery sessions, including several technical sessions on radial turbomachinery aerodynamics, microturbines, turbochargers, and oil and gas applications. www.asme.org
The European Turbomachinery Conference. This is organised every two years by the European Turbomachinery Society, which represents many engineering associations from most countries in Europe and includes technical sessions on axial, radial and mixed-flow turbomachinery. www.euroturbo.eu
The International Conference on Turbochargers and Turbocharging. This is organised by the Institution of Mechanical Engineers, England, and addresses current and novel aspects of turbocharging system designs. www.events.imeche.org
The Turbomachinery and Pump Symposium. This is organised annually by the Texas A&M University, Houston, and is an industry event, offering a forum for the exchange of ideas between rotating equipment engineers and technicians worldwide.
https://tps.tamu.edu/event-info/
The American Institute of Aeronautics and Astronautics (AIAA) Science and Technology Forum and Exposition. The AIAA SciTech Forum is an important annual event for aerospace research, development and technology bringing together aerospace industry experts in a variety of technical disciplines
The major journals which include publications on centrifugal turbocompressors are the following:
The Journal of Turbomachinery, ASME. The Journal of Turbomachinery publishes high-quality, peer-reviewed technical papers that advance the state of the art of turbomachinery technology, including gas-path technologies associated with axial compressors, centrifugal compressors and turbines.
https://turbomachinery.asmedigitalcollection.asme.org/journal.aspx
The Journal of Engineering for Gas Turbines and Power, ASME. The Journal of Engineering for Gas Turbines and Power publishes high-quality papers in the broad technical areas of gas and steam turbines, internal combustion engines and power generation including centrifugal compressors.
https://gasturbinespower.asmedigitalcollection.asme.org/journal.aspx
The Journal of Power and Energy, Institution of Mechanical Engineers (IMechE). The Journal of Power and Energy, Part A of the Proceedings of the Institution of Mechanical Engineers, is dedicated to publishing papers of high scientific quality on all aspects of the technology of energy conversion systems.
https://journals.sagepub.com/home/pia
International Journal of Rotating Machinery, Hindawi. The International Journal of Rotating Machinery is a peer-reviewed, open access journal that publishes original research articles as well as review articles on all types of rotating machinery.
www.hindawi.com/journals/ijrm/
The Journal of Propulsion and Power, AIAA. This journal provides readers with primary interests in propulsion and power access to papers spanning the range from research through development to applications in fluid dynamics and combustion, including many turbocompressor papers.
https://arc.aiaa.org/jpp/about
International Journal of Turbomachinery, Propulsion and Power (IJTPP). This is an international peer-reviewed open access journal focused on turbomachinery, propulsion and power. It is the official dissemination tool of Euroturbo, the European turbomachinery society, which runs the European Turbomachinery Conferences.