Introduction
Fluorescence microscopy techniques are now prevalent throughout the life sciences and many of the physical sciences. These techniques are often dependent on white light sources that have evolved from the more traditional mercury arc lamp to metal halide sources to the more recent light emitting diodes (LEDs). The newer light sources show more uniform power across the visible light spectrum, allowing for the use of fluorophores and fluorescent proteins outside of the peak wavelengths associated with the more traditional light sources.
These developments have led to considerable choice on the fluorescence light source market, and so a number of questions have arisen: Is it necessary to replace traditional, but trusted, mercury arc lamps? Is photobleaching or phototoxicity an issue with your current light source? Which light source should be used for which applications? These questions must be answered before making a choice from the plethora of light sources available on the market. This article reviews the pros and cons of several light sources and discusses their uses for specific applications.
What Does Your Fluorescence Microscope Need to Do?
To produce a high-quality quantitative microscopy image, three things need to be achieved: (1) The image of the specimen must be magnified relative to the size of the original object, (2) there must be enough contrast in the image to distinguish the details within the specimen from its surroundings, and (3) there must be sufficient resolution to distinguish between different objects or features within the specimen. All three of these aspects must be realized in order to generate quantitative fluorescence images (Figure 1).

Figure 1: A microscope needs to generate an image of the specimen that is magnified, has high resolution, and optimal contrast. Images were collected of Hoechst nuclear stain (blue), paxillin-EGFP labeled focal adhesions (green), and tubulin antibody stain (red) using a mercury lamp. (A) High magnification and resolution, but not enough contrast. (B) High magnification and contrast, but not enough resolution. (C) High contrast and resolution, but not enough magnification. (D) Ideal image with high magnification, contrast, and resolution.
Mercury Arc Lamps
Mercury arc lamps are still found in most research labs. They are often designated by the registered trademark “HBO”. H is short for the element mercury (Hg), B is the symbol for luminance, and O is the symbol for unforced cooling. These are high-powered white light sources that generate many intense bands for fluorescence excitation across the UV-visible light spectrum (http://zeiss-campus.magnet.fsu.edu/articles/basics/ fluorescence.html). In fact, many of the traditional dyes were selected to have absorption peaks that specifically corresponded to the mercury spectral peaks for optimal fluorescence excitation. Mercury arc lamps have the advantage of producing a lot of power at these spectral peaks, as well as being readily available. This light source also covers the entire visible spectrum; if a new dye is required for a certain application, a filter cube can be purchased at a reasonable cost and used in conjunction with the lamp. One of the disadvantages of mercury arc lamps is a non-uniform illumination across the microscope field of view (Figure 2A and 2B). Because the light originates from a concentrated arc, bulb alignment is required each time the bulb is changed (newer models have more straightforward alignment procedures). Furthermore, the bulbs have to be replaced every 200–300 hours, the lamp intensity decays over time, and the bulbs contain mercury that must be disposed of as a hazardous waste. For live cell imaging, neutral density (ND) filters must be used to reduce photo bleaching [Reference Frigault, Lacoste, Swift and Brown1] and photo toxicity. Although a large component of the mercury lamp spectrum is ultra-violet (UV) light, UV blocking filters should be used to minimize UV leakage to living samples. This is because the blocking by dichroics and filters is typically not strong enough to block all of the UV radiation and allow the relatively low-intensity fluorescence signals through. However, this UV component is ideal for the excitation of dyes such as Fura. Mercury arc lamps can be used to collect high-quality quantitative fluorescence images. Note that because of sample heterogeneity, it is difficult to visualize the non-uniformity in the image field (Figure 2C), but this effect should still be corrected for [Reference Lacoste, Young, Brown, Taatjes and Roth2].
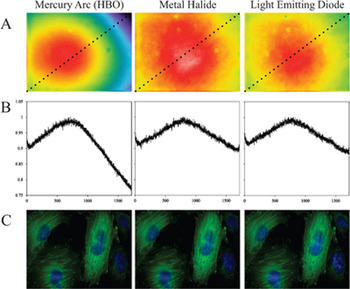
Figure 2: Field illumination intensities and fluorescence images. Images were taken on a widefield microscope with an EGFP fluorescence cube on a scientific-grade CCD camera with no pixel binning. (A) Images of a yellow-green fluorescent slide in order to observe the excitation field illumination uniformity. (B) The pixel intensities of a diagonal line across each image from A, measured using a 3-pixel average. The intensity data was normalized to the maximum intensity for each image to compare the field uniformity for the three light sources. (C) Images of the same fixed CHO (Chinese hamster ovary) cells, stably expressing paxillin-EGFP and stained with DAPI, were imaged with each light source. Images were background-corrected, processed with a “sharpen” filter, and a gamma factor was applied to emphasize dim features. Scale bar is 20 μm. Images in A are the same size as images in C.
Xenon Arc Lamps (XBO)
Xenon arc lamps have very similar pros and cons to mercury arc lamps. However, they have much more uniform excitation intensities across the visible spectrum, albeit at lower powers (http://zeiss-campus.magnet.fsu.edu/articles/basics/fluorescence.html). The bulb intensity still decays over time, but the bulbs have a longer lifetime of ~1000 hours. Xenon arc lamps are a true white light source accommodating any dye of choice across the UV, visible and infrared (IR) spectrum. Xenon lamps deliver a lot of power in the IR spectral region, which is ideal for IR excitable dyes, but heat filters need to be put in place for live cell imaging.
Metal Halide Lamps
Metal halide lamps have a very similar spectrum when compared to mercury arc lamps, except that the peak intensities are slightly lower, and the intensities between the peaks are significantly higher. These properties provide more uniform excitation intensity across the visible spectrum (zeiss-campus.magnet.fsu.edu/articles/lightsources/metalhalide.html). Metal halide bulbs cost more than twice as much as HBO bulbs, however they last up to 10 times as many hours (~2000). These lamps are pre-aligned, and they deliver light via a liquid light guide and microscope adaptor, ensuring uniformity of illumination across the field of view (compare Figure 2A and 2B). In addition, the remote coupling of the lamp also reduces heat at the microscope and allows for remote shuttering of the lamp, reducing potential vibrations of the microscope during time-lapse imaging. Metal halide lamps generate high-quality fluorescence images (Figure 2C), and a key advantage for quantitative imaging is that the intensity of the bulb is more stable over time.
Light Emitting Diodes (LEDs)
LEDs seem well positioned to take over the market as the fluorescence light source of choice [Reference Wessels, Pliquett and Wouters3]. Homemade versions [Reference Cole and Turner4] have been around for about 10 years, whereas commercial systems have become available over the last 5–6 years. LEDs are compact and can be built into basic microscope stands. Each LED offers a discrete excitation peak and can be independently and rapidly switched on and off within milliseconds. This minimizes the need for excitation shutters and filters. It should be noted, however, that LEDs in the yellow and green region of the spectrum are fairly broad, covering a range of wavelengths, and they often require some filtering to avoid fluorophore excitation cross-talk. LEDs have a lifetime on the order of 10,000 hours, and they do not generate as much heat as mercury, xenon arc, or metal halide lamps. They do not require any warm-up or cool-down time, the intensity can be precisely controlled, and intensity does not decay over time. They also do not contain mercury, which reduces the amount of hazardous waste relative to mercury or xenon bulbs. Early in their development, the number of wavelengths was limited, and the power of the LEDs was disappointing. However, there are now LED sources ranging from the UV to the near IR range, and higher-power LEDs are also available. Some LED light sources are coupled directly to the microscope, whereas the majority are in a remote combiner, which contains the LEDs and optical elements necessary for combining and filtering the various colors. The LED source is connected to the microscope with a liquid light guide providing a more uniform excitation across the field of view (Figure 2A and 2B). Many systems now contain a number of LEDs, allowing them to essentially mimic white light sources. High-quality images comparable to the mercury arc and metal halide light sources can be generated with LEDs (Figure 2C). LEDs are not true white light sources so new LEDs may need to be purchased when using new or novel dyes.
An Example LED Light Source
The X-Cite® XLED1 is the latest LED light source available from Lumen Dynamics, Mississauga, Canada (www.ldgi-xcite.com). Each LED is guaranteed for 20,000 hours or 3 years, and the system can be programmed for time-lapse, sequential, or multi-color imaging. The LED light source is attached to the microscope by a liquid light guide and a microscope coupler and can be controlled using the touch-screen control panel or a computer software interface (Figure 3A). Drivers are currently available for Image-Pro Plus (Media Cybernetics, Inc.), MetaMorph® (Molecular Devices, LLC), Micro-Manager (Open Source), Nikon NIS-Elements (Nikon Instruments Inc.), and SlideBook™ (Intelligent Imaging Innovation, Inc.). The system can be equipped with 4 of the available 13 LEDs spanning from the UV to the IR (Figure 3B). The 4 LED wavelengths can be chosen using the online custom configuration application (http://www.ldgi-xcite.com/products-xled1-configurator.php). For example, LEDs at 405 nm and 460 nm can be chosen for the shorter wavelengths, and 525 nm and 635 nm LEDS can be chosen for the longer wavelengths. These 4 LEDs would be ideal for blue (e.g., DAPI), green (e.g., Alexa-488 or EGFP), red (e.g., Alexa 555 or RFP) and far red dyes (e.g., Alexa 647). The configuration application will determine the positions of these LEDs and specify which dichroics are required for any given combination of LEDs (Figure 3C). More than 4 LEDs can be purchased with the system, and the LEDs and associated optics are user-interchangeable. However, it should be noted that only 4 LEDs can be used at one time, so if novel dyes or novel dye combinations are used, new modules must be added.

Figure 3: The X-Cite® XLED1 light source. (A) Light source with the liquid light guide, microscope coupler, and the control touch pad. (B) Normalized spectra of the various LEDs currently available from Lumen Dynamics. (C) Schematic of the LED coupling optics for the 405, 460, 525, and 635 nm LED.
LEDs for Live Cell Imaging
LED light sources are ideal for multi-color, live cell imaging. The LED intensities can be precisely controlled within ~0.1%–1% accuracy, and there is no UV or IR component to the spectra, thus there is no need for UV or heat filters. Of course, UV or IR wavelength LEDs can be chosen for dye excitation. Depending on the light sensitivity of the sample, ND filters may still be required. There is also no need for shutters because each LED can be switched on and off independently. For the highest sensitivity, it is best to use separate fluorescence filter cubes for each dye to be imaged. However, for rapid live cell assays, a multiband dichroic mirror and a multiband emission filter can be used with the rapid switching of the LEDs providing dye selectivity. However, when using multiband mirrors and filters, it is crucial to run controls with single labeled samples in order to correct for excitation and emission cross-talk (http://www.microscopyu.com/articles/fluorescence/fret/fretintro.html).
Power Comparison
It can often be difficult to compare the power across different light sources. This is because power is usually expressed in terms of watts for the total output of the light source across all available wavelengths. However, the power is spread over a number of wavelengths, and the physical size of the light sources can vary. Therefore, a 100-watt mercury HBO and a 100-watt xenon lamp have very different power distributions. For example, the mercury HBO has a lot of its power in the UV part of the spectrum, whereas the xenon lamp has a lot of its power in the IR region. LEDs may have much lower wattage, but they typically only span a very small part of the spectrum. Therefore, it is best to express or ask for power specifications in terms of the spectral irradiance. That is the power in milliwatts (mW) per unit area of the light source per nanometer (nm) of wavelength, or the power density per nm (http://zeiss-campus.magnet.fsu.edu/articles/lightsources/lightsourcefundamentals.html). Only then can accurate comparisons between light sources be made. This is why a 25 mW laser that is specific for a single wavelength can be focused to a small spot and will have a much higher power density then a 100-watt xenon bulb at that same wavelength.
Light Source Comparisons
Table 1 shows a summary of the pros and cons of various light sources for quantitative, live cell, and high-speed imaging. Although LED light sources may have a higher upfront cost, their low maintenance, intensity stability, long lifetime, and rapid switching can make them an ideal choice for fluorescence imaging. It is important to remember that LEDs are not a true white light source, and depending on the light source design, they may need to be interchanged or the system may need to be upgraded with new LEDs if new dyes or dye combinations are to be used. This is not the case for white light sources such as mercury, xenon, or metal halide. If a new dye is to be used, one simply has to purchase a new filter cube with the appropriate filters and dichroic mirror for the given dye. When using multiple band dichroics and filters, make sure to add appropriate controls and correct for fluorophore cross-talk. All of the light sources perform well and can be used to generate high-quality, quantitative fluorescence images (Figure 2C).
Table 1: Pros and cons of light source technologies for quantitative, live cell, and multi-color imaging.

Conclusions
Conventional lamp-based technologies (mercury, xenon, metal halide) are still useful for many applications and have the benefit of being truly white light sources. Nevertheless, if lamps are used 8 hours a day or more, it may be worth doing a cost-benefit analysis (for assistance see http://www.ldgi-xcite.com/products-calculator.php). Taking into account the staff time to change and align the bulbs and the cost of the bulbs themselves, replacing them with an LED-based system may pay for itself within a year or two. However, if the lamp is only used for a few hours a day and field non-uniformity is corrected, then traditional light sources can still be very useful and economical. For more information on light sources, watch the recording of the webinar “LAMP? LED? LASER? Which light source is best for your microscopy application?” by Claire M. Brown at http://www.ldgi-xcite.com/news-webinars.php.
Acknowledgments
The images in the paper were collected and this work was supported by the McGill Life Sciences Complex Imaging Facility. Images in Figure 1 were taken by Kimberly Young. Tushare Jinadasa and Claire M. Brown declare no financial interest in Lumen Dynamics.