Introduction
In 1994, silicon (Si) is introduced as a microwave substrate in characterizing the coplanar transmission line [Reference Reyes, El-Ghazaly, Dorn, Dydyk and Schroder1], and then after 5 year in 1999, the first integrated dipole antenna is designed to study the feasibility of antennas on Si substrates [Reference Kim and Ko2]. After that, an unparalleled development in designing on-chip antennas (OCAs) is noticed for the wide frequency spectrums starting from lower microwaves (1–30 GHz) [Reference Radiom, Mohammadpour-Aghdam, Vandenbosch and Gielen3–Reference Guclu, Sloan, Pan and Capolino7] to millimeter (mm)-waves (30–300 GHz) [Reference Babakhani, Guan, Komijani, Natarajan and Hajimiri8–Reference Baek, Truong, Park, Lee, Chae, Rhee, Park and Rhee10] and terahertz (THz) bands [Reference Sankaran, Mao, Seok, Shim, Cao, Han, Arenas, Tanner, Hill, Hung and O11–Reference Park, Kang and Niknejad13].
However, the progressive evolutions of very large-scale integration (VLSI) circuit technology drove to integrate the complete electronic system on a single chip, which in turn is known as system-on-chip (SoC). The SoC technology enables integration of all the functional modules of the transceiver such as digital baseband and RF front-end on a single chip, and results in achieving the goal of realizing the most demanding features of a compact system with reduced power consumption and cost [Reference Cheema and Shamim14]. However, an antenna being the largest component, still remains outside of the chip and increases the overall size of the transceiver system. Inclusion of the antenna along with other functional modules in the same chip provides several advantages in addition to benefits of SoC mentioned above. For reference, making an off-chip antenna and bringing the same to connect with other parts of the transceiver system requires the constraint of maintaining the same port impedance, which is usually 50 Ω. However, OCA and the other circuitries of the transceiver can be designed together on the same substrate. This gives the flexibility of matching an arbitrary value of the antenna impedance (Z A = R A + jX A) with its complex conjugate (R A − jX A) by changing other parameters from the remaining part of system components. Thus, the inherent issue of matching the antennas’ port impedance with the specific value of the transceiver or feeding port impedance is vanished. Furthermore, it removes the requirements of costly flip-chip connections between the integrated circuits and the off-chip antenna.
A typical application of OCAs integrated on SoC is demonstrated in Fig. 1. The introduced OCAs are used for short range communications like inter-chip and intra-chip communication, wireless clock distributions, RFID's communication, etc. Usually, the complete signal transmission links were carried out through the wire connections as shown in Fig. 1(a). Interconnections of large circuit components are made through thin conducting path. In the highly denser integrated circuits, the interconnecting network becomes too complex. Also, because of transmission delay through the thin conduction path, it does not support the present requirement of higher data rate (≈GBs). Finally, but not the least, several interconnecting thin wires produce severe attenuation losses as the frequency of operations increases [Reference Yordanov and Russer15]. Figure 1(b) illustrates a similar configuration of Fig. 1(a), just by replacing the wired-connections with wireless connections using OCAs integrated on the same chip. The inclusion of OCAs in the SoC devices provides multiple leverages like, low latency rate, reliable communication, less transmission losses, reduced complexity of communication network, and significant reduction of area due to the absence of wires which makes the device more compact. Also, the antenna along with the other circuitries of the device can be monolithically implemented on the same chip. Thus, the OCA provides an overall technological edge to the SoC devices in terms of data carrying capacity, low latency, and compactness by fulfilling the users’ requirements.

Fig. 1. An example of on-chip communication.
However, implementation of antennas on a chip leads to multiple design challenges. First, the integration of antenna becomes difficult due to its large size, especially for lower microwave operating frequencies. Although antenna miniaturization techniques [Reference Volakis, Chen and Fujimoto16] can be applied to make its size compatible to chip, reduction of antenna's aperture reduces the gain as well as radiation efficiency. Second, to the VLSI industry, Si is the first choice as a substrate for integrating circuit. In contrary, low resistivity (ρ ≈5–10Ω cm) and high permittivity (εr = 11.9) of Si are not suitable for antennas. By considering a simple patch antenna, its working principle is illustrated in Fig. 2. The antenna radiates both toward air and substrate. The low value of ρ governs ohmic loss and a part of the energy is wasted in the form of thermal energy which results in increased substrate temperature. The high εr attracts and couples the electro-magnetic (EM) wave to produce surface waves within the substrate instead of radiation in the free-space as shown in Fig. 2(b). As an outcome, with reduced gain and efficiency, it radiates less in air as shown in Fig. 2(c).

Fig. 2. OCA with Si as substrate: (a) top view of a CPW-fed patch antenna; (b) EM wave radiation; and (c) radition output and substrate loss.
Generally, OCAs are used for short range applications like intra-chip and inter-chip [Reference Floyd, Hung and O17–Reference Jiang, Mao and Yin19], short range radar [Reference Pepe, Vallozzi, Rogier and Zito20, Reference Kucharski, Ahmad, Ng and Kissinger21], RFID tags [Reference Chen, Yeoh, Choi, Li and Singh22, Reference Radiom, Baghaei-Nejad, Aghdam, Vandenbosch, Zheng and Gielen23], biomedical implantable devices [Reference Le, Fong and Luong24, Reference Rodrigues, Gonçalves and Mendes25], etc., where along with the efficient radiation characteristics, low power, compact system, capable in fast data communication is the prime concern. Moreover, the present and upcoming generation of the communication networks such as 5G and 6G demand more traffic handling capacity, reduced latency, and less energy consumption. To achieve such targeted performance, design of efficient antenna on Si by overcoming associated hindrance is required. A brief overview regarding performance improvement of OCAs is discussed in [Reference Mandal, Mandal and Mal26], however, the discussion is limited to the use of artificial magnetic conductors (AMCs) thus required a detailed review with potential future scopes.
This paper demonstrates some important design challenges associated with OCAs and their trending solutions along with some perspective future applications. Section “Prime challenges in OCA” displays the important role of the substrate and various methods for designing an efficient antenna. The developments made toward improvement of OCA performances are explored in Section “Remedies.” The immense potential future applications of OCAs are highlighted in Section “Future scope.” Finally, the overall conclusion is drawn in Section “Conclusion.”
Prime challenges in OCA
Limitation of the antenna on Si
Most of the semiconductor industries preferred Si-based complementary metal–oxide–semiconductor (CMOS) technology for the fabrication of ICs. This is because the thermal, electrical, and mechanical properties of Si are perfectly suitable for IC design. As an OCA needs to be integrated along with the other circuit components of ICs on a single chip, the Si as substrate becomes obvious choice for designing antenna despite of its unsuitability.
To study the antenna performance on Si, a conventional quarter-wave monopole antenna is designed by considering perfect electric conductor (PEC) as a ground surface of the antenna [Fig. 3(a)] and its performance is compared with the monopole antenna where silicon substrate is used as ground as shown in Fig. 3(b). The EM simulation software, high frequency structure simulator (HFSS) is used to study the antenna performance.
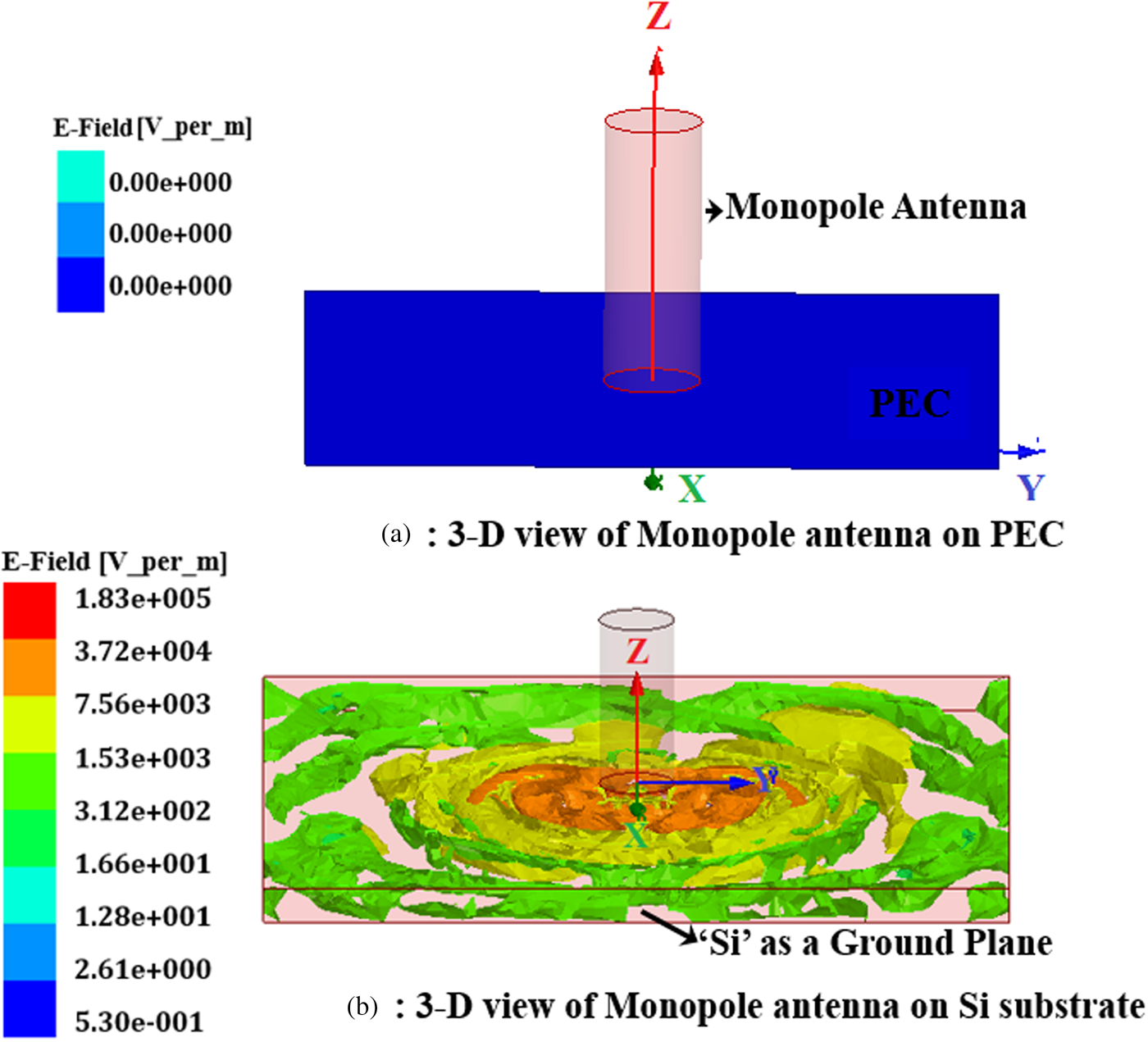
Fig. 3. Study of E-field distribution on PEC and Si ground cases for vertical monopole.
For the case of grounded PEC, the current distribution is uniform throughout the thin surface of the PEC and, as expected, the magnitude of the current is zero. It is because, a part of the monopole antenna radiated field is incident on the conductor surface and the same is completely reflected back toward the upper side. Therefore, in the upper half of the radiation zone, the resultant field is obtained with the superposition of the direct and reflected fields while there is no radiation in the lower half. Thus, no power dissipation is observed through the grounded PEC surface as shown in the Fig 3(a).
On the other hand, the traditional micro-fabrication technology deals primarily with Si as a substrate material targeting the batch fabrication process and precise lithographic structure realization. Inherently, Si is a lossy dielectric material for which the antenna performance, specifically, efficiency and subsequently the gain of antenna is affected if the antenna is made it. Figure 3(b) shows that significant current is distributed within the Si substrate when a monopole antenna is designed on it. This is because, in contrast to PEC, Si is not able to reflect the incident EM wave completely. As a result, some part of EM waves resided within the substrate due to relatively higher value of dielectric constant and form surface waves and some part of the penetrated EM wave power is transmitted from the bottom surface of the substrate. Furthermore, because of finite resistivity, contact of the radiator with the silicon substrate at the feed/excitation point causes a major current sinking within the dielectric material. Therefore, a significant amount of input power fed to the antenna is wasted when the antenna is designed on Si substrate even with a separate PEC layer as ground of the antenna. For reference, the wastage of power becomes worst when a printed monopole or dipole antenna is designed on silicon substrate. According to [Reference Babakhani, Guan, Komijani, Natarajan and Hajimiri8], for a dipole antenna designed on Si, 97% of the power is coupled with the Si and only 3% is radiated into air. This wastage of power within the substrate leads to reduce the antenna efficiency and hence the antenna gain.
These studies indicate unsuitability of Si as an antenna substrate and a quest for its alternatives is required. In this regard, gallium arsenide (GaAs) [Reference Baek, Truong, Park, Lee, Chae, Rhee, Park and Rhee10, Reference Chen, Chiou and Chen27, Reference Passiopoulos, Nam, Georgiou, Ashtiani, Robertson and Grindrod28] and silicon germanium (SiGe) [Reference Neculoiu, Muller, Tang, Laskin and Voinigescu29, Reference Ojefors, Sonmez, Chartier, Lindberg, Schick, Rydberg and Schumacher30] are used and because of their high resistivity as compared to Si substrate, an improved characteristics of OCA is investigated. However, these substrates are used in specific applications due to their limited availability and higher cost.
Limitation of design rule check
In the process of IC design, it is important to verify the design rules for each component before consideration of a specific design layout. This is because, all individual foundries have their own certain set of rules to ensure good fabrication maintaining tolerance limit and design repeatability. Among the hundreds of design rules, some specific rules such as metal density in each layer of the CMOS layout, thickness of metal and oxide layers with spacing between them, and maximum number of vias with dimensions, need critical attention in the designing of OCA [Reference Cheema and Shamim14]. In general, for any semiconductor foundry, the allowable metal density for a given layer should be between 30 and 70%. The thickness of the top metal layer wherein OCA is usually designed is limited to 20 μm. However, such metal thickness is not suitable to achieve efficient radiation characteristics of the designed OCA, particularly at LMF range. Thus, it is always recommended to analyze the DRC for the feasibility of realizing the designed prototype by reducing the possible fabrication errors.
Requirement of miniaturization at lower frequencies
As per Moore's law [Reference Moore31], the integration of circuit components on a fixed footprint area of a chip becomes double in every 2 years. This continuous advancement in the VLSI technology enables to produce the size of the IC-based end products much smaller than ever. On the other hand, the standard antenna size should be λ0/2 for its optimum performance. Here, λ0 is the free-space wavelength. Now, for higher operating frequency (over 30 GHz at mm-wave, sub-mm-wave, and THz bands), the normal λ0/2 antenna is small enough to accommodate within a chip. However, the actual problem appears at lower frequencies (below 30 GHz) where the size of antenna becomes significantly large and antenna miniaturization essentially required to fit within a comparatively smaller chip area. Miniaturization is a process where the electrical length of antenna is increased without affecting its physical length in such a way that the operating frequency shifts toward the lower side. However, this process directly affects the antenna aperture subsequently the gain and radiation efficiency as these are directly related to the aperture size. Thus, one has to always make a compromise with the antenna performance and higher miniaturization so as to accommodate the antenna within the smaller chip area by fulfilling the desired radiation characteristics for the intended applications.
Remedies
The major challenge of designing an OCA with efficient radiation characteristics is to overcome the substrate loss. In this regard, it is essential to reduce the substrate coupling of EM wave. Thus, several techniques, based on the introduction of the dielectric resonator, AMC, micro-electromechanical systems (MEMS), micro-machining, and partially shield layers, focus primarily on reducing substrate loss. As far as the design rule is concerned, the standard CMOS technique is followed in most of the reported studies. The primary reason behind is that it provides a fixed set of rules to antenna designers as well as integration compatibility of the antenna along with various other IC components. However, this leads to select Si as a substrate for the antenna. Various methods that are used to reduce the substrate loss are discussed in the following section.
Application of dielectric resonator
The successful deployment of upcoming 5G and 6G communication networks at mm-wave frequency require small size, high gain, and efficient antenna. The microstrip or the printed circuit planar antenna techniques have several advantages: low cost, light-weight, and easy integrability with the system chip. However, because of the conduction loss associated with metal at higher frequencies and the substrate loss for the significant coupling of the fringing fields with the Si, the efficiency of such antenna becomes poor. Elsewhere, dielectric resonator antennas (DRAs) are usually made up of lossless dielectric materials such as ceramics which makes them less lossy compared to the metallic counterparts at higher operating frequencies. DRAs characterized with relatively higher dielectric constant reduce substrate loss by reducing the fringing fields and become a suitable candidate for designing high gain and efficient OCAs at mm-waves, sub-mm-waves, and THz frequency ranges [Reference Hou, Hong, Goh, Chen, Xiong, Hu and Madihian32–Reference Singh, Mandal and Karmakar39]. The dielectric constant of DR material is an important parameter and it mainly controls the antenna characteristics. Moreover, DRAs are equally applicable for wide frequency spectrum. The DRA is usually placed on the top of planar antennas as shown in Fig. 4 or in some cases, mounted with the non-planar antennas [Reference Singh, Mandal and Karmakar39, Reference Wu, Kodi, Kaya, Louri and Xin40]. Because of higher permittivity compared to air, it enhances the directive gain of the antenna [Reference Guha, Banerjee, Kumar and Antar41]. Also, in some cases, the DR materials themselves act as radiators when directly fed with the excitation probe [Reference Singh, Mandal and Karmakar39, Reference Thakur, Dwari, Kanaujia and Pandey42]. However, at LMFs the planarity is lost with the comparatively larger size of DR and some special care is required for the packaging of the three-dimensional (3D) structure. The state-of-the-art reported OCAs using DRA is listed in Table 1. It shows that the achieved antenna gain is less due to miniaturization at LMF [Reference Burasa, Djerafi, Constantin and Wu33, Reference Singh, Mandal and Karmakar39] while OCAs designed at sub-mm wave offer sufficient gain without requirements of any miniaturization [Reference Deng, Li, Liu, Wu and Xiong35–Reference Ali, Yun, Ng, Kissinger, Giannini and Colantonio38]. The problem of gain reduction due to miniaturization can be sorted out by operating DRA at higher-order modes. In [Reference Hou, Hong, Goh, Chen, Xiong, Hu and Madihian32], the feed point of DRA is optimized to shift the higher-order modes – TEδ13, TEδ15 modes and sufficient gain of 6.2 and 7.5 dBi are respectively achieved with an OCA size of λ0/5.5. Another important aspect of the designing OCAs using dielectric resonators is observed in [Reference Singh, Mandal and Karmakar39] where, without any metal layer of CMOS stack, Si substrate is used as ground for the antenna and DRA is used to increase the directive gain and efficiency by 12.3 dBi and 41% respectively. Usually, another intermediate metal layer is utilized as a reflector/ground to minimize the effect of substrate loss [Reference Zhu, Li, Feng, Xiao and Zhang43–Reference Nafe, Syed and Shamim45]. However, in the proposed antenna in [Reference Singh, Mandal and Karmakar39], the DR material with high permittivity and high resistivity confines most of the fields and allows maximum radiation toward air instead of substrate and thus eliminates the requirement of the metal layer for reduction of substrate losses.
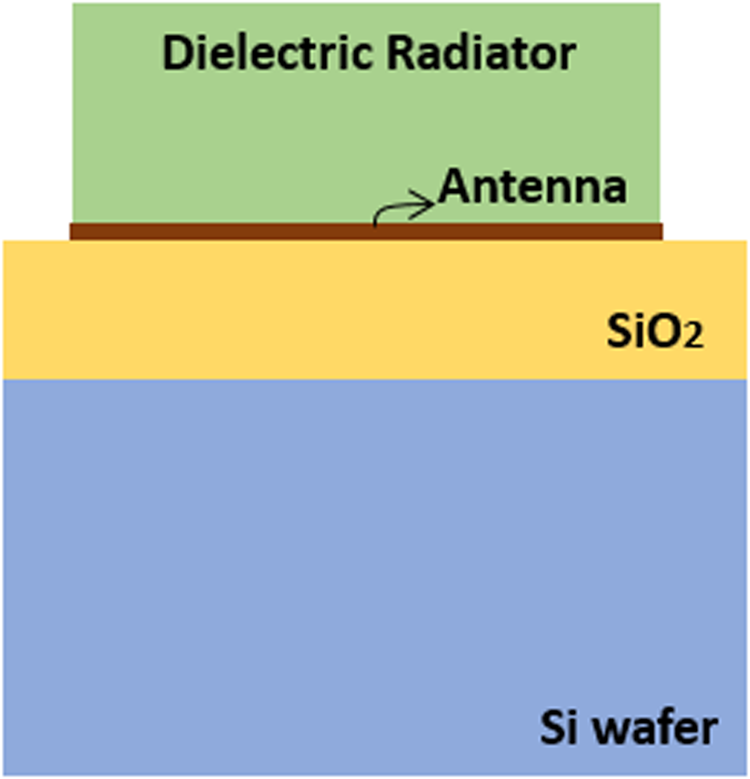
Fig. 4. An on-chip dielectric resonator antenna.
Table I. Current state-of-the-art in on-chip DRA

d = maximum dimension of antenna, G = gain (dB), η = efficiency (%), BW = bandwidth.
Introduction of artificial magnetic conductors
In an OCA, the primary reason of low gain and efficiency is due to the coupling of the EM fields with the substrate. In this regard, AMCs can be judiciously used to isolate the antenna from the lossy Si. Table 2 shows the few recent developments on the OCA using AMCs. AMC presents the properties of perfect magnetic conductor. It is to be noted that, use of PEC reflects the waves out of phase, creates destructive interference and results in decreased antenna efficiency. On the other hand, AMC exhibits zero degree phase reversal at resonance. This zero phase reversal of the waves produces constructive interference and contributes to enhanced radiation performance of the antenna. Different techniques to employ the zero phase reversal theory of AMC have been introduced for enhancing the radiation performance of OCAs [Reference Zhu, Li, Feng, Xiao and Zhang43–Reference Yang, Cui, Ding and Lv47, Reference Lan, Guo and Zhang49]. A two-layered dipole OCA (connected using vias) is fabricated with a 14 × 7 unit cells of AMC ground and the gain enhancement of 5 dBi with 63% of efficiency is observed [Reference Zhu, Li, Feng, Xiao and Zhang43]. An AMC is designed using artificial engineered superstrate meta-material to improve OCA characteristics in [Reference Zhang and Shamim44]. The superstrate and lens allow radiation along a particular direction and provides highly directive gain. The AMC works as a shield as well as reflector for the EM waves and prevents propagation toward Si. In [Reference Liu, van den Biggelaar, Johannsen, van Beurden and Smolders46], an additional layer of AMC is implemented to mitigate the dielectric losses significantly. It [Reference Yang, Cui, Ding and Lv47], an OCA gain of 9.8 dB is achieved by using AMC in the intermediate between top layer antenna and substrate, and off-chip-based ground below the Si wafer. This structure is improved for SoC devices in [Reference Nafe, Syed and Shamim45], where both ground and AMC layers have been shifted above the Si wafer as shown in Fig. 5. It can be seen that the both AMC and ground in the proposed structure act as the reflector as well as shield for EM waves to propagate toward lossy Si. Thus, AMCs ensure highly improved radiation performance of OCAs. However, an additional metal layer for AMC ground plane increases an extra step during fabrication process and thus twofold increased in the design complexity.
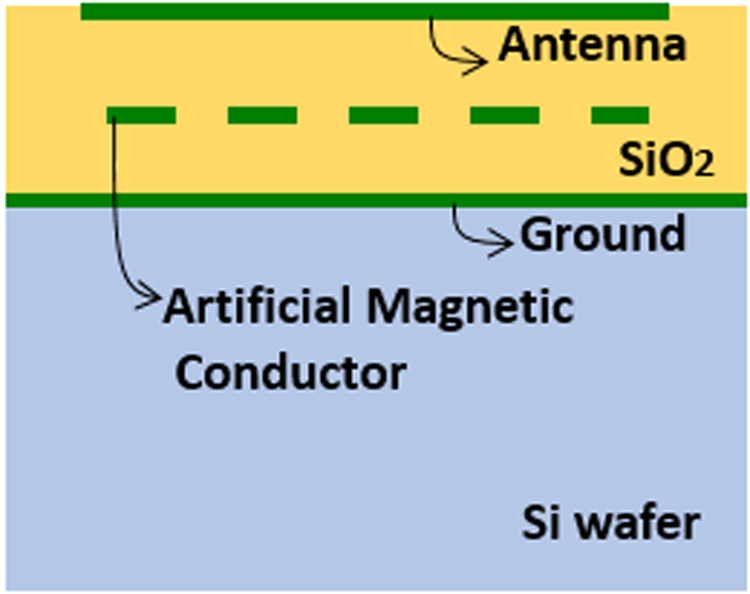
Fig. 5. An OCA with AMC.
Table 2. Current state-of-the-art in OCA using AMCs

f r = resonating Frequency (GHz), G = gain (dB), d = max. dimension in terms of free-space wavelength.
Incorporation of MEMS-based technology
It is already mentioned that the high dielectric constant and low resistivity of Si causes poor antenna radiation characteristics. Introduction of MEMS isolates the OCAs from the lossy silicon substrate mechanically. An MEMS technology is defined as a miniaturized system which includes both mechanical and electronics components. Usually, MEMS technique allows us to design cantilever, arch, suspension, or beam-like antennas on substrates (as depicted a cantilevered OCA in Fig. 6). The radiation pattern of these type of antennas are tilted at certain angle depending upon the bending of the cantilever structure. These type of antennas are useful for beam scanning and beam tilting applications [Reference Kesavan, Mantash, Zaid and Denidni48]. The separation from the lossy substrate made them an efficient one in comparison with conventional planar antennas. Table 3 shows the list of reported OCAs using MEMS technology in the last 10 years. The technique is used to design a composite right/left handed transmission line (RLH TL) based miniaturized OCA in [Reference Lin, Chang and Hsieh50]. The composite RLH TL is used to realize miniaturization as well as zero phase constant near resonant frequency. This gives zeroth order resonance regardless of frequency, which consequently helps for implementing compact circuit design. In [Reference Marnat, Ouda, Arsalan, Salama and Shamim51], MEMS-based frequency reconfigurable OCA is proposed and fabricated. The cantilevered prototype of OCA can be oriented on both E- and H-planes from a fixed position by using hinges and operated at 59 and 66 GHz resonance frequencies. Using MEMS technology, a self-assembled 200 μm wide and 1250μm long cantilever structured monopole antenna with improved performance is proposed and fabricated in [Reference Lee, Chen, Titz, Ferrero, Luxey, Vaughan and Parameswaran52]. Unlike OCDRA, for which size constraints its application at lower frequencies, MEMS technology offers applicability for wide range of frequencies from lower microwave to THz band. An OCA with high gain is developed for UWB applications at 3.2–11.9 GHz [Reference Xiaoming, Baisen and Xiaoguang53] whereas a 3D helical structure is designed for 4 THz frequency band using MEMS [Reference Guo, Meng, Zhang and Ge54]. However, the design complexity of such antenna is increased awfully and, accordingly these are recommended for specific application-based devices.
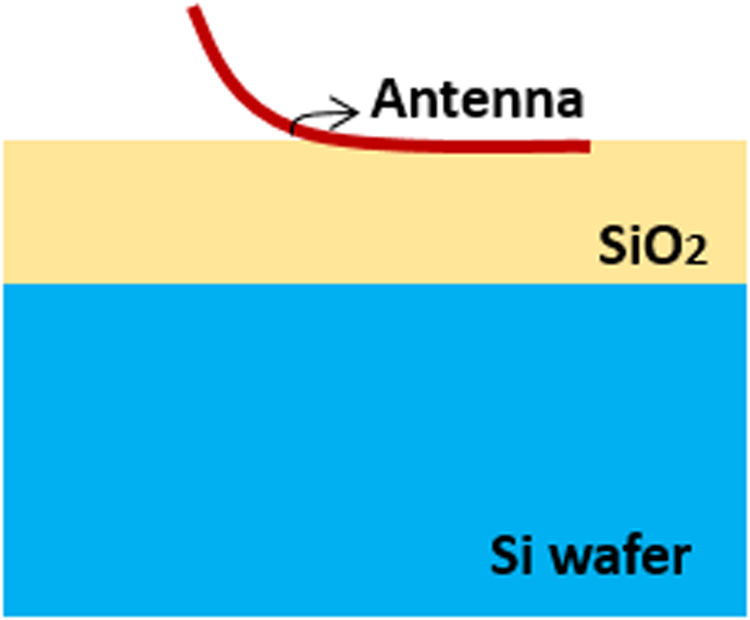
Fig. 6. A cantilevered on-chip monopole antenna designed using MEMS technology.
Table 3. Current state-of-the-art in OCA using MEMS-based technology

f r = resonating frequency (GHz), G = gain (dB), d = max. dimension in terms of free-space wavelength.
Micromachining of Si wafer
Micromachining is appeared as a promising technology for improving OCA characteristics. The technique is used to remove the redundant part of the higher refractive indexed, lossy Si from bottom surface of the substrate as shown in Fig. 7. The localized etching technique is used to remove a redundant part of the backside Si appearing underneath the radiating antenna. The vacant part is filled with the low loss dielectric medium having a lower refractive index such as air (ɛrSi = 1) or polyamideqtoa “polymide” has been changed to “polyamide”. Please check. (ɛrSi = 3.2). The removal of Si wafer and then filling the vacant space with air leads to reduce effective dielectric constant as well as substrate loss and, subsequently improves radiation characteristics of the antenna. It is investigated that the antenna performance can be improved further by introducing high resistive material in the vacant cavity created by micromachining the substrate. The developments of OCAs in the last 10 years using micromachining technology is shown in Fig. 8. In [Reference Chu, Guo, Lim, Khoo and Shi55], where an addition of benzocyclobutene and another polymer material in micromachined cavity helps to reduce substrate loss and achieve a gain of 6.4 dB with a miniaturized λ0/3.7 size of OCA. 3D micro-machining is another complex but effective technique to characteristics enhancement of OCAs. In [Reference Somjit and Oberhammer56], to design a complete integrated antenna with high gain of 13.2 dB, 3D micromachining is performed. In this W-band antenna, an air-cored helical structure is designed using multiple level of metal layers connected through vias and integrated into the Si wafer. Similarly, other high gain OCAs have been reported in recent years for higher microwave and mm-wave frequency applications using micromachining are depicted in Fig. 8 [Reference Khan, Ç. Ulusoy, Dufour, Kaynak, Tillack, Cressler and Papapolymerou57–Reference Sallam, Serry, Sedky, Shamim, Vandenbosch and Soliman62]. It is worth mentioning here that the advantage of micromachining is that it can be implemented along with the other gain enhancement techniques as performed in [Reference Sallam, Serry, Sedky, Shamim, Vandenbosch and Soliman62]. In [Reference Sallam, Serry, Sedky, Shamim, Vandenbosch and Soliman62], the micromachined on-chip DRA provides a high gain of 9.44 dB and 97% of efficiency. Thus, to reduce losses due to Si, micromachining technique provides a tool to remove the significant amount of lossy Si. Another significant advantage with this technique is that it is a post fabrication process and thus no fabrication complexity increases. However, removal of substrate has to be optimized to maintain the mechanical strength of the OCA and overall device.

Fig. 7. An OCA designed using back-side micromachining.

Fig. 8. Current state-of-the-art in OCA using micromachining in recent years.
Inclusion of dielectric and metal layers
Some of the techniques like incorporation of partial shield layer (PSL) [Reference Singh, Mandal, Mandal and Karmakar63, Reference Singh and Mandal64], thin film of hafnium oxide [Reference Singh and Mandal65], ferrite loading [Reference Singh and Mandal66, Reference Singh, Mandai and Mandai67] in standard CMOS structure of OCA are recently reported successfully for enhancement of radiation characteristics. Connecting the top layered antenna with PSL through a metal via increases the overall electrical path length and offers antenna miniaturization. The PSL not only provides miniaturization by increasing electrical length but it also acts as a shield for the propagation of EM wave toward lossy Si and reduces the substrate losses. A layer of HfO2 (ɛr ≈ 21–27) over the SiO2 layer refracts the EM waves toward outer side for radiation instead of Si and enhances the radiation efficiency. The ferrite loading as shown in Fig. 9 is another good technique to improve the performance of OCA significantly. The thin film inclusion in between the metal and SiO2 completely isolates the radiating metallic layer from Si wafer and thus reduces substrate loss. Along with that, the ferrite layer adds an extra reactive element in antenna circuitries which shifts the resonance toward a further lower operating frequency and helps to miniaturize the OCA as well.

Fig. 9. Inclusion of a thin ferrite layer into standard OCA's layout.
Other techniques
There are many other techniques like silicon lens [Reference Park and Wong68], ion irradiation [Reference Hirano, T Inoue, Yagi, Okada and Matsuzawa69], asymmetrical patch [Reference Singh, Hazarika, Kumar and Mandal70], impure Si wafer (high resistivity Si) [Reference Jou, Azadegan and Mohammadi71, Reference Lin and Ooi72], and split ring resonators (SRRs) [Reference Mustacchio, Boccia, Arnieri and Amendola73] are some of the popular and trending techniques recently, which have been reported for enhancing the radiation characteristics of OCAs. In [Reference Park and Wong68], a rectangular-shaped Si lens is used to increase gain by 8–13 dB. The PSL not only provides the miniaturization by increasing electrical length but also acts as shield for the propagation of EM wave toward lossy Si [Reference Singh, Mandal, Mandal and Karmakar63]. Ion irradiation in [Reference Hirano, T Inoue, Yagi, Okada and Matsuzawa69] leads to less losses from Si wafer and subsequently enhancement in gain is observed. The asymmetry in the patch's slot changes the radiation of antenna and improves the directive gain [Reference Singh, Hazarika, Kumar and Mandal70]. Similarly, high resistivity Si wafer doesn't absorb much EM waves and reduces losses as reported in [Reference Jou, Azadegan and Mohammadi71, Reference Lin and Ooi72, Reference Pan, Wang, Guclu and Capolino74]. In [Reference Mustacchio, Boccia, Arnieri and Amendola73], the SRRs geometry and their coupling with the master antenna can be set in such a way that they create an additional resonance that cancels gain drops. Thus, these techniques provide improved radiation characteristics of OCAs. However, the requirement of miniaturized and efficient OCA for SoC applications always brings challenges for antenna researchers.
Future scope
As the 5G and 6G generation technologies are continuously demanding smaller systems, on-chip antennas are going to be very significant in terms of providing chip level compactness. This also opens many area for researchers to explore and utilize their knowledge of designing miniaturized antenna for small SoCs. Some of them are given below.
Adequate use of emerging substrates
Over the years, the semiconductor market is mostly relies on the use of substrates like SiGe, GaAs, SiC, and InP [Reference Cheema and Shamim14]. Parallelly, the growing industries have started to explore the opportunities of utilizing some emerging substrates like galium–antimony compound (GaSb), indium–antimony compound (InSb), galium nitrate (GaN), galium oxide (Ga2O3), aluminum nitrate (AlN), etc. [Reference Developement75]. These emerging substrates have higher resistivity (e.g. the electrical resistivity of InP and GaAs material in the order of 106–108 Ω cm, million times better than that of Si), which provides excellent isolation and substrate insulation and subsequently results better radiation characteristics to OCAs. However, such emerging wafers are expensive and their low cost alternatives are in development process. According to a market study report by Yole Development, these emerging substrates are going to cover more than 200% of its current market in upcoming years [Reference Developement75]. Thus, it opens new area of research for the antenna designers to analyze the electrical and thermal properties of these engineered substrates and provide efficient OCAs with improved radiation characteristics.
OCA in space application
The latest advancements in space-based devices such as nano-satellites, pico-satellites, femto-satellites, etc. [Reference Sweeting76] have opened another vital area for the OCA applications. The evolution of small satellites leads to many emerging applications like radar and sensors [Reference Gao, Rahmat-Samii, Hodges and Yang77], telemetry, surveillance [Reference Najati, Basari, Rahardjo and Zulkifli78], hardware testing, academic operations and laboratories [Reference Sweeting76, Reference Pittella, Pisa and Nascetti79], land deformation detection [Reference Sri Sumantyo and Imura80], weather foresighting [Reference Phong, Manh, Khac and Chien81], and many more. Generally, a satellite contains different modules like control system, power supply, communication system, payload, mechanical system, etc. A communication system is the pivotal part and many small conventional antennas are utilized for intra and inter-satellite communication. As the miniaturization is the prime requirement of these systems, OCAs bring compactness to the overall system. Using the novel engineered semiconductor substrates [Reference Developement75] and continuous emergence of novel techniques for enhancing radiation characteristics promises the efficient OCAs for these applications.
Massive MIMO technology
The present communication technology is going to shift toward the 5G and 6G networks for which compact devices supporting high speed data transfer, low latency rate, and reliable transmission are required. Multiple input multiple output (MIMO) and massive MIMO technology is used to fulfill these requirements [Reference Malviya, Panigrahi and Kartikeyan82–Reference Ishteyaq and Muzaffar84]. The massive MIMO-based 5G and 6G devices consist of around hundreds antennas in their RF front-end. The separate feed network of such higher number of antenna elements by providing proper impedance matching becomes complex and needs higher space. MIMO system with OCAs may alleviate the requirement of separate feed network with additional constraint of impedance matching. Thus, use of OCAs may be the ideal choice for the MIMO architecture to make a compact and economical system for the future generation networks. There are few works have reported on OCA-based MIMO systems [Reference Jing, Rowell, Raju, Chan, Murch and Yue85, Reference Ng and Kissinger86]. A folded dipole on-chip MIMO antenna fabrication and its measurement has been demonstrated in [Reference Jing, Rowell, Raju, Chan, Murch and Yue85]. Another highly miniaturized folded dipole along with radar sensor is designed for angular measurements using MIMO networks in [Reference Ng and Kissinger86]. Still, the future generation networks demand highly miniaturized on-chip MIMO antennas which pave the another interesting and vibrant research area of MIMO OCAs.
THz devices applications
Typically, the frequency spectrum between 300 GHz and 3 THz is defined as the THz band. The design of antenna for the THz communication network is one of the most attractive areas of research as it offers large bandwidth for high speed data communication operating at less crowded spectrum. Meanwhile, there are some hindrances in terms of high path loss in the free-space and limited range of coverage. However, in the THz band, the major advantage of OCA is the size of the antenna becomes comparable to the normal IC chip. As an outcome, even without any miniaturization the antenna can be accommodated and the realized antenna can provide good radiation characteristics [Reference Gao, Du, Zhang and Guo87, Reference Saxena, Manur, Mansoor and Ganguly88]. In [Reference Saxena, Manur, Mansoor and Ganguly88], the graphene-based on-chip antenna array has been employed to empower energy-efficient, multi-modal inter—chip communication protocol for multichip systems in the THz range. Thus, OCAs may play an important role for the upcoming networks in the THz range.
Biomedical applications
In the last two decades, the increased in-vivo implant reliability with demand of low-miniaturized electronics devices steered the biomedical instrumentation world toward the development of smaller wireless electronic devices, such as bladder pressure monitoring [Reference Majerus, Garverick, Suster, Fletter and Damaser89], intelligent gastric and cardiac pacemakers [Reference Cheng and Tereshchenko90, Reference Bolz91], implantable pacemakers and defibrillator [Reference Boveda, Garrigue and Ritter92], and deep brain stimulator [Reference Winfree, Pretzer-Aboff, Hilgart, Aggarwal, Behari and Agrawal93]. The Medical Implant Communication System utilizes some fixed frequency bands such as (MICS-415 MHz band), ISM 915 MHz, 2.45 GHz band) for transmitting data of implantable devices. The normal antenna size operating at this frequency band is larger and subsequently, this results in large device. Implantation of large device requires painful invasive surgical procedure and should be avoided. Thus, the miniaturized OCA emerged as a solution for the smaller system. However, miniaturization comes at the cost of reduced gain and efficiency. Usually, the implantable devices are used in the short range and high data rate communication with far field gain of − 30 dBi and efficiency between 1 and 5% are adequate [Reference Kiourti and Nikita94, Reference M. Huang, -R. Tofighi and Rosen95]. Thus, OCAs become suitable for implantable biomedical applications. Nevertheless, implantable antennas required some pre-requisite design conditions such as human body likewise simulation and characterization environment. Moreover, the antenna must follow two other critical parameters to become suitable for any implant applications namely Biocompatibility and specific absorption ratio (SAR) limit test. In order to make a system biocompatible, an OCA needs to be simulated with a layer of biocompatible materials like Teflon (ɛr = 2.1, tanδ = 0.001), ceramic alumina (ɛr = 9.4, tanδ = 0.006), Si3N4 (ɛr = 7, tanδ = 0.005) [Reference Kiourti and Nikita94]. For SAR test, IEEE C95.1-1999 standard suggest that, the average SAR level at or below 1.6 watts per kilogram (W/kg) taken over the volume containing a mass of 1 g of tissue that is absorbing the most signal [Reference Cheema and Shamim14, Reference Kiourti and Nikita94]. Although, many OCAs for biomedical devices have been reported [Reference Marnat, Ouda, Arsalan, Salama and Shamim51, Reference Singh and Mandal65, Reference Jou, Azadegan and Mohammadi71, Reference Masius and Wong96, Reference Karacolak, Hood and Topsakal97] still, the improvement of radiation performance under implant environment is much needed. However, the continuous demand of miniaturized implant devices keep motivates researchers for delivering user friendly and small implant devices.
Conclusion
OCAs are found to be beneficial in wide range of applications as detailed in this paper. The research on this field is still in the flourishing stage. Therefore, it manifests several research opportunities such as the new design concepts based on improving antenna performances, the optimum position of the antenna in the system with increased radiation characteristics by minimizing power loss, and the effect of radiated EM wave with other circuit components, investigation of the novel antennas for the upcoming modern communications. Along with substrate losses, significant miniaturization of the OCA in the low frequency significantly affects its radiation performances. Hence, the prosperity of OCA is more suitable in the mm-wave and Terahertz communications. Also, it will play a vital role in achieving a completely compact RF-SoC system.
Harshavardhan Singh obtained B.E. in electronics and communication engineering (ECE) from NRI-Institute of Information Science and Technology, Rajiv Gandhi Proudyogiki Vishwavidyalaya Bhopal, India in 2010, and obtained M.Tech. in electronics and communication engineering (telecommunication engineering) from National Institute of Technology Durgapur, India in 2014. He joined the institute as a research scholar in 2015 to pursue a Ph.D. degree. He received scholarship from the Visvesvaraya Ph.D. scheme, The Ministry of Electronics and Information Technology, Government of India during his research study. Currently, he works as an assistant professor in the Department of Electronics and Communication Engineering, University of Engineering and Management, India. His primary area of research is on-chip antennas, system-on-chip (SoC)-based architectures, chip inductors, antenna arrays, and RF circuits for wireless communication. He has published 16 research papers in International Journals and Conferences.
Sujit Kumar Mandal obtained his B.Sc. in physics honours from the University of Calcutta in 2001. He completed his B.Tech and M.Tech in radio physics & electronics from the Institute of Radio Physics & Electronics, C. U. in 2004 and 2006, respectively. He obtained a Ph.D. degree from the National Institute of Technology, Durgapur where he is presently an assistant professor in the Department of Electronics and Communication Engineering. His current research area includes application of soft computing techniques in antenna array optimization, time-modulated antenna arrays, microstrip patch antennas, RF-energy harvesting, and on-chip antenna design. Dr. Mandal is a member of IEEE.