Introduction
Purified rod-shaped frustules of diatoms are characterized by typical patterns consisting of small lamellate dark and bright stripes that are equidistant from each other. The smallest spaces are about 0.2 μm or less; such small distances correspond with the usual limit of resolution in light microscopy. Therefore, high-end equipment is necessary for the observation and photomicrography of these fine structures. In this paper, we report on the lens and illumination combinations required for good results in this special task.
Materials and Methods
Some permanent slides (stewed slides) were used for all observations containing several diatom shells: Navicula sp., Surirella gemma, Nitzschia sigmoidea, Nitzschia obtusa, Frustula rhomboidea, and Amphipleura pellucida. These specimens differ in their size and the lattice constants (typical distances) of their subcellular bright and dark stripes (Figure 1, Table 1). Within the respective frustules, the bright stripes result from small perforations separated in very short distances that cannot be resolved in light microscopy. The neighboring dark stripes correspond to small zones that are not perforated (Figure 2).
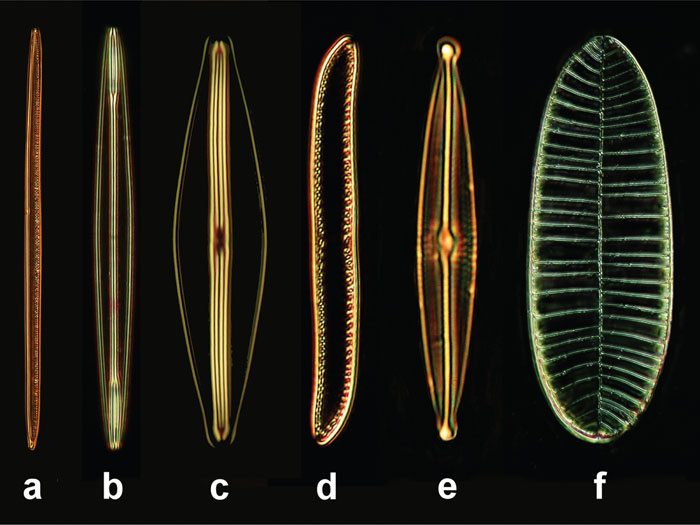
Figure 1: Diatoms selected for our examinations, objective 40×, length spec-ification in μm. (a) Nitzschia sigmoidea (220 μm), (b) Amphipleura pellucida (80 μm), (c) Frustula rhomboides (50 μm), (d) Nitzschia obtusa (60 μm), (e) Navicula sp. (50 μm), (f) Surirella gemma (100 μm).
Table 1: Morphological data of diatom shells selected for our optical tests[Reference Göke6]


Figure 2: Formation of bright and dark stripes caused by linear patterns of small perforations (further explanations in the text).
Visual observations were carried out with high-end lenses from Carl Zeiss: Neofluar 40/0.75, Planapo 40/1.0, and Planapo oil 100/1.3. For bright-field illumination, a compatible high-end achromatic-aplanatic condenser was used; its numerical aperture was 1.4, and its head lens group could be used as a dry system or with oil-immersion.
For photomicrographs, two special lenses were used: a water immersion 120/0.9 designed by Carl Zeiss Jena as a catadioptric mirror system and an ultra-high magnifying lens from Leitz/Leica (Planapo Oil 160/1.4). These lenses were both combined with a standard universal condenser for bright-field and phase contrast illumination (dry system, numerical aperture 0.9). The annular light masks within this condenser could be used for eccentric oblique illumination when they were turned in a moderately off-centered position. Moreover, a dark-field condenser, designed as an immersion system, numerical aperture 1.2, was used together with the 160× magnifying lens in order to achieve a bright-field-like concentric oblique illumination. By use of the mirror lens, axial dark-field illumination (luminance contrast) was carried out (optical path in Figure 3); the principle of this illumination mode has already been reported [Reference Piper1, Reference Piper2].

Figure 3: Light path of axial dark-field illumination (luminance contrast), catadioptric mirror lens, yellow: illumination light, red: imaging light, ad = aperture diaphragm, ss = specimen slide, cs = cover slip.
The illuminating light (halogen light and LED Luxeon Star for white light) was filtered by monochromatic narrow-band filters, which are also used in astronomy: green light (λ = 540 nm), blue-green light (λ = 500 nm), and blue light (λ = 486 nm). The half-intensity width of these filters ranges from 8.0–8.5 nm (technical data compiled in Table 2). Moreover, the monochromatic green filter was compared with a 540-nm LED. For this task, specimens were examined with the 540-nm LED and in white light filtered with the 540-nm narrow-band astronomy filter, and the resulting images were visually compared with each other. Some general findings about the use of the astronomy filters mentioned above have already been described in a previous article [Reference Piper3]. Photomicrographs were taken with a 7.1-MP digital camera (Olympus Camedia C-7070) fitted with a 12.5 fold magnifying eyepiece (Leitz/Leica vario-photo-ocular turned into the highest magnification).
Table 2: Optical data of monochromatic astronomy filters used for our evaluations

Results
When the 40-fold magnifying Neofluar lens was used, only the patterns within Navicula sp. could be detected in white light (lattice constant 0.66 μm). When illuminated by the shortest wavelength (λ = 486 nm), the smaller patterns from Nitzschia sigmoidea were barely visible (lattice constant 0.33 μm). By using the 40-fold magnifying oil immersion, the patterns from Nitzschia sigmoidea also were barely visible in white light and appeared in higher sharpness and contrast when illuminated with monochromatic light. All smaller patterns (lattice constants <0.33 μm) were not visible in 40-fold objective magnification.
By using the 100-fold magnifying oil immersion, the stripes within Nitzschia sigmoidea became barely visible in white light, but all smaller patterns could not be recognized as long as the illuminating light was unfiltered. In monochromatic green light (λ = 540 nm), visible improvements in image quality were just moderate and primarily apparent in Navicula sp. To observe the smaller patterns, the illuminating light had to be filtered in blue-green (λ = 500 nm) or blue light (λ = 486 nm). The blue-green light produced the highest contrast and led to the most differentiated presentation of all tonal values. Blue light (λ = 486 nm) promised the highest resolution, but the image contrast was sometimes lower than in blue-green light.
In white light illumination, the condenser aperture diaphragm had to be turned into a moderately closed position to visualize the respective patterns. When the diaphragm was wide open, the image contrast was too low for all lenses, and all patterns remained invisible in white light. On the other hand, the condenser diaphragm could remain wide open when monochromatic filters were used.
The very small patterns in Amphipleura pellucida could only be observed at an adequate resolution and contrast when the light was filtered in blue and the condenser was used in immersion mode. In the other species, an immersion of the condenser head lens group did not lead to visible enhancements in image quality. Also in all white-light observations, the image quality was not influenced in a visible manner by use of oil immersion on the condenser head lens.
The 540-nm LED was not suitable for enhancements of the image quality. Resolution, contrast, and sharpness were comparable with normal light illumination. As a reason for this finding, it could be taken into account that the half-intensity width of the LED was not as small as in the corresponding monochromatic astronomical filter (see the section “materials and methods” and Table 2).
The results achievable with the 160-fold magnifying apochromatic oil immersion lens are demonstrated in Figures 4–8. Amphipleura pellucida was the only species that had to be illuminated by an immersion condenser (dark-field condenser, numerical aperture 1.2, bright-field-like concentric oblique illumination) and had to be imaged in monochromatic light (λ = 500 nm or 486 nm) in order to see the linear pattern. In this specimen, blue light led to superior resolution (Figure 8). In all the other species, the normal universal condenser (dry system, numerical aperture 0.9) was capable of visualizing the respective linear patterns (Figures 4–7). Also in this lens, the final resolution, sharpness, and contrast could be enhanced by monochromatic light in the same way described above. In most cases, the resolution could be optimized further when a suitable annular light mask within the universal condenser was turned in an off-centered position to obtain an eccentric oblique illumination (Figures 6 and 7).

Figure 4: Navicula sp., objective Planapo oil 160/1.4, bright field, universal condenser (dry system, numerical aperture: 0.9), line spacing: 0.66 μm, illumination with unfiltered white light, monochromatic green (540 nm) and blue (486 nm) light.

Figure 5: Surirella gemma, objective Planapo 160/1.4, bright field, condenser from Figure 4, line spacing: 0.33 μm, illumination with unfiltered white light, monochromatic green (540 nm) blue-green (500 nm) and blue (486 nm) light.

Figure 6: Nitzschia sigmoidea, objective Planapo 160/1.4, condenser from Figure 4, eccentric oblique illumination, line spacing: 0.33 μm, illumination with unfiltered white light and monochromatic green light (540 nm).

Figure 7: Nitzschia obtusa, objective Planapo 160/1.4, condenser and illumination from Figure 6, blue light (λ = 486 nm), line spacing: 0.20 μm, illumination with monochromatic blue light (486 nm), conversion in black and white.

Figure 8: Amphipleura pellucida, objective Planapo 160/1.4, immersion condenser for dark field (numerical aperture: 1.2), concentric oblique illumination, line spacing: 0.20 μm, illumination with monochromatic blue-green (500 nm) and blue (486 nm) light, conversions in black and white.
Navicula sp. could also be examined and photographed very well with the mirror lens (120×/0.9) based on axial dark field (luminance contrast). By this illumination technique, the axial resolution (depth of field) could be maximized. Also in this case, sharpness and resolution were improved by monochromatic light, whereby blue-green light (λ = 500 nm) lead to the best results (Figure 9).

Figure 9: Navicula sp., specimen from Figure 4, mirror lens, water immersion 120/0.9, axial dark field (luminance contrast), illumination with unfiltered white light and monochromatic blue-green (500 nm) light, conversions in black and white.
Discussion
When small linear patterns have to be examined or photographed in diatom shells, there are three important technical factors to consider to achieve excellent image quality. The most important factor is a lens with high numerical aperture, high magnification, and high-end correction.
The second factor is the use of monochromatic narrow-band filters. In most cases, monochromatic green light (λ = 546 or 540 nm) leads to the lowest enhancement of quality when compared with shorter wavelengths; the respective improvements of quality are primarily visible in moderately spaced patterns (Navicula sp., for instance). Blue-green light (λ = 500 nm) promises better results, especially in small patterns; the final resolution is visibly higher than in 546- or 540-nm wavelength, the enhancement of contrast is maximized, and all nuances of tonal values are accentuated in a superior manner when compared with the other filters. Blue light (λ = 486 nm) should be used for visualizations of extremely small patterns, which correspond to the general limit of resolution (for example, Amphipleura pellucida), but the remaining contrast may be lower than in 500-nm light. The positive effects of monochromatic narrow-band filters are much higher than those resulting from immersion condensers. Moreover, only monochromatic filters lead to substantial enhancements of contrast while the condenser aperture diaphragm can be wide open for observations of ultra-fine low-contrasted structures. All in all, the 500-nm filter might be recommended as a universal filter leading to the best results in most cases. When compared with white light, the resolution increases at least by about 15% with the help of 500-nm or 486-nm filters.
Oblique illumination is the third factor that can contribute to enhancements of lateral resolution. Universal condensers for phase contrast are well-suited for this task. Also dark-field condensers with a high numerical aperture can be used effectively for concentric oblique illumination in bright-field, as long as the numerical aperture of the objective is higher than that of the condenser. According to theoretical considerations, the resolution can be doubled when switching from axial illumination to extreme oblique illumination [4].
In contrast to monochromatic narrow-band filters, so-called monochromatic green LEDs (nominal wave-length: 546 nm) do not produce improvements over normal white light illumination. When the condenser is used in immersion mode, no visible improvements can be expected in most cases (exception: Amphipleura pellucida). Each monochromatic filter promises better results than any immersion of the condenser. Also very small patterns (for example, Nitzschia obtusa, lattice constant 0.20 μm) can be visualized successfully with a high-quality dry condenser (NA 0.9) in oblique short-wavelength monochromatic light. Our recommendations for all technical applications described above are compiled in Table 3.
Table 3: Technical guidelines for visualizing ultra-fine patterns in diatoms

In light microscopy, image resolution can be enhanced further when specimens are illuminated in ultraviolet light. Very impressive images taken in ultraviolet light—also from Amphipleura pellucida and Surirella gemma—are presented on the web by Höbel [Reference Höbel5]. The resolution achievable in ultraviolet light is superior to all techniques presented here, but ultraviolet illumination cannot be used for visual observations with the human eye. Thus, the techniques presented in this paper lead to the highest resolution in visible light, which can be used for normal visual examinations.
Of course, these procedures also can be used for examinations of other transparent structures with repeating fine details near the microscope resolution limit. The diatom frustules presented here were examined and photographed as particularly good examples for the optical improvements resulting from our methods.
Conclusion
This contribution deals with the highest resolution that can be achieved based on conventional light microscopy using visible light. Specific recommendations of lenses and illumination conditions are presented.